DOI:
10.1039/C5RA25792A
(Paper)
RSC Adv., 2016,
6, 15745-15752
Hydrothermal synthesis of a Ba and Mg co-doped Bi12GeO20 photocatalyst with enhanced visible light catalytic activity
Received
3rd December 2015
, Accepted 26th January 2016
First published on 28th January 2016
Abstract
Visible-light-activated (Ba,Mg)-codoped Bi12GeO20 has been successfully synthesized through a one-step hydrothermal method. The as-prepared samples were characterized by X-ray diffraction (XRD), field-emission scanning electron microscopy (FE-SEM), high-resolution transmission electron microscopy (HRTEM), X-ray photoelectron spectroscopy (XPS), UV-vis diffuse reflectance spectra (DRS) and photoluminescence spectroscopy (PL). XPS study suggests that barium and magnesium were introduced into the Bi12GeO20 crystal successfully. The band gap of (Ba,Mg)-codoped Bi12GeO20 was greatly reduced in comparison with the pure and single-doped Bi12GeO20. (Ba,Mg)-codoped Bi12GeO20 showed higher photocatalytic activity than pure Bi12GeO20 and single-doped Bi12GeO20 in the photodegradation of Rhodamine B (RhB) aqueous solution under visible light irradiation. Photogenerated holes were the dominating active species, with the secondary and minor factors of hydroxyl radical and superoxide radical in the photodegradation process of (Ba,Mg)-codoped Bi12GeO20. For three cycles, the co-doped Bi12GeO20 exhibited high stability.
1. Introduction
Since Fujishima and Honda1 reported the photocatalytic splitting of water on TiO2 electrode,2,3 extensive research has been done in the synthesis and application of TiO2 based photocatalysts. However, owing to its large energy band gap (3.0–3.2 eV), TiO2 is only responsive to ultraviolet light, which only accounts for 5% of the solar spectrum.4–7 Moreover, the high recombination rate of photogenerated electron–hole pairs in TiO2 results in quite low quantum efficiency (1%).8 Therefore, it is necessary to explore visible-light-induced photocatalysts with high efficiency.
Bi12GeO20 belongs to the sillenite group of cubic crystals with the general formula Bi12MxO20±δ (M = Ti, Si, Ge).9,10 It has been identified as promising applications in photorefractive materials, capacitors and ferroelectric materials, due to its relevant electrical and optical properties. Recently, Bi12GeO20 has attracted considerable interest for its great photoconductivity and optical absorption, which fit the essential demand for an effective photocatalyst. The unique body-centred cubic structure with an MO4 tetrahedron in the centre makes it convenient for Bi12GeO20 to dissolve the novel metal ions,11,12 because the tetrahedron of oxygen atoms surrounding the M atom is able to expand or contract under the premise of not greatly affecting the remaining atomic arrangement.13 Meanwhile, alkaline earth metal elements in the second main group of the periodic table have two electrons in the outermost electron shell, which tends to lose two electrons to form a full shell structure. This full-shell electrons configuration allows the alkaline earth metal ions as dopants to change the electronic structures of the host.14 At the same time, no gap states and electron–hole recombination centres are introduced in the process. Besides, regardless of dopant ionic radius and charge, doped metal ions always replace the position Ge of Bi12GeO20 tetrahedral centre.14 Yao et al. reported that doping with Ba2+ significantly increased the photoactivity of Bi12TiO20 crystals.13 They also found that the Ba2+ took the place of Ti4+, thereby forming oxygen vacancies for the charge compensation. Hence, the co-doped Bi12GeO20 with alkaline earth metal is an effective and promising way to enhance the photocatalytic activity.15–17 To the best of our knowledge, alkaline earth metal co-doping Bi12GeO20 has never been studied.
Herein, (Ba,Mg)-codoped Bi12GeO20 was prepared via a one-step hydrothermal method for the first time. The photocatalytic activities of the as-prepared samples were evaluated by the photodegradation of Rhodamine B (RhB) under visible light irradiation. The effects of different doping amounts on the photocatalytic activities were discussed in details and the degradation mechanism was studied as well.
2. Experimental
2.1. Preparation of materials
All the chemicals were of analytical purity and directly used without further purification. Undoped and doped Bi12GeO20 powers were synthesized via a one-step hydrothermal method. Ba(NO3)2 and Mg(NO3)2 metal salts were selected as barium and magnesium sources respectively. Co-doped Bi12GeO20 crystals were prepared as follows: 12 mmol Bi(NO3)3·5H2O and 1 mmol GeO2 were dissolved into 70 mL alkaline solution containing 5 mol NaOH. Then desired amount of Ba(NO3)2 and Mg(NO3)2 aqueous solution was added dropwise to the solution under magnetic stirring. The mixture was vigorously stirred to disperse all reagents homogeneously for one hour. The resulting mixture was transferred into a 100 mL stainless steel Teflon-lined autoclave for hydrothermal treatment at 180 °C for 12 h, and cooled to room temperature naturally. The resultant precipitates were filtered and washed with deionized water and absolute ethanol several times. Finally, the products were dried in a vacuum oven at 60 °C for 12 h. The dopant concentrations mentioned in this work are the nominal atomic concentration, which is based upon the assumption of quantitative incorporation of the dopant.
2.2. Characterization
The X-ray diffraction (XRD) measurement was performed on a Bruker D8 ADVANCE X-ray diffractometer equipped with Cu-Kα radiation. Field-emission scanning electron microscope (FE-SEM, IEISS Ultra 55, Germany), operated at accelerating voltages between 20 and 30 kV, was employed to observe the morphology of the sample. The microstructures of as-prepared samples were investigated by high-resolution transmission electron microscope (HRTEM, JEM 2100HR, JEOL, Japan) with an accelerating voltage of 200 kV. The Brunauer–Emmett–Teller (BET) surface area of samples was measured through nitrogen adsorption (Micromeritics ASAP 2010). The photoluminescence (PL) spectra were measured at room temperature using a fluorescence spectrophotometer (Hitachi, F-2700). The surface chemical composition of products was detected by X-ray photoelectron spectroscopy (XPS, ESCALAB 250, Thermo-VG Scientific, USA). UV-vis diffuse reflectance spectra were obtained by using a Hitachi UV-3010 spectrophotometer with BaSO4 as a reflectance standard.
2.3. Photocatalytic test
Photocatalytic activities of the as-prepared samples were evaluated by degradation of RhB aqueous solution under visible light irradiation. All the photocatalytic reaction was carried out in the XPA photochemical reactor (Nanjing Xujiang Machine-electronic Plant, China) at room temperature as follows: 0.5 g photocatalyst was added into 100 mL RhB aqueous solution with an initial concentration of 5 mg L−1. Before irradiation, the suspension was stirred magnetically in dark for 0.5 h to achieve adsorption–desorption equilibrium on the catalysts. Subsequently, the reactor was irradiation by a 500 W Xe arc lamp with a 420 nm cutoff filter. At given time intervals, about 3 mL suspension was sampled and filtered through 0.45 μm membrane filters for analysis. The concentration of the filtered RhB was analyzed by UV-vis 8500 spectrophoto-meter (Shanghai Tianmei Science Apparatus Ltd. Co. China).
3. Results and discussion
3.1. Characterizations
3.1.1. XRD analysis. The phase composition and crystallographic structure of pure and doped Bi12GeO20 were studied by XRD measurements. As shown in Fig. 1, the sharp and strong diffraction peaks indicate the as-prepared samples have good crystallinity. All the characteristic diffraction peaks are in good agreement with the standard XRD patterns of the Bi12GeO20 (JCPDS no. 34-0096) and no impurity diffraction peaks were detected. Furthermore, the characteristic peaks of Ba-, Mg-, and co-doped Bi12GeO20 showed the slight shift. The lattice parameters of the samples were obtained (Table 1) according to Bragg's law and the formula for a cubic system, |
λ = 2d sin θ
| (1) |
|
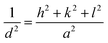 | (2) |
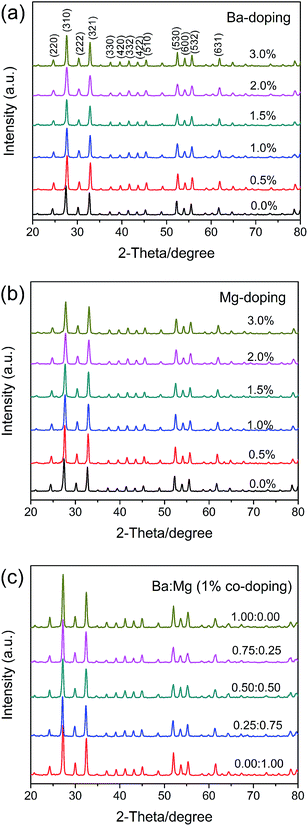 |
| Fig. 1 The XRD patterns of the as-synthesized products: (a) 0.0–3.0% Ba-doped BGO; (b) 0.0–3.0% Mg-BGO; (c) 1% (Ba,Mg)-codoped BGO. | |
Table 1 Band gaps (Eg) and pseudo-first-order rate (k) constants of different samples
Sample |
Lattice parameters (a) |
BET (m2 g−1) |
Eg (eV) |
k (min−1) |
Pure BGO |
10.146 |
8.21 |
2.37 |
0.0886 |
1% Ba-BGO |
10.177 |
44.87 |
2.06 |
0.1838 |
2% Mg-BGO |
10.153 |
32.54 |
2.25 |
0.1308 |
1% Ba/Mg-BGO |
10.212 |
86.69 |
1.93 |
0.4979 |
The lattice parameters increased with the introduction of doping ions, which indicated the possible incorporation of Ba and Mg ions into the structure of Bi12GeO20. With respect to the codoping, the peak shift and intensity of the XRD spectra did not vary significantly when the total doped dosage was invariable. It is worth noting that no characteristic peaks about Ba, Mg metal oxides were observed, which may be due to the low content of Ba and Mg compound, or the high dispersion in the Bi12GeO20 crystals.18,19
3.1.3. UV-vis DRS analysis. The band gap structure of semiconductor plays an important role in the photocatalytic activities. UV-vis diffuse reflectance spectra of as-prepared samples were shown in Fig. 4. The band gap energy (Eg) of the as-synthesized samples can be calculated by the following equation: |
 | (3) |
where Eg is the band gap energy (eV) and λ is the wavelength (nm) of the absorption edge. The band gap of pure Bi12GeO20 was calculated to be 2.37 eV. It is clearly observed that all the as-prepared samples have considerable optical absorption under visible light and the absorption edges of doped Bi12GeO20 show slight red-shift. These results proved that the doped ions successfully entered into the Bi12GeO20 and had an effect on UV-vis absorption characteristics of Bi12GeO20 crystal. The red shift of absorption towards longer wavelength was further enhanced as the concentration of doped Ba2+ or Mg2+ increased.14 The incorporation of doped ions formed new impurity energy level between the valence band and the conduction band, improving the utilization ratio of visible light.20 The band gap of Ba-doped Bi12GeO20 is slightly narrower than that of Mg-doped Bi12GeO20, while that of the co-doped Bi12GeO20 was narrowest. For instance, the band gaps are calculated to be 2.06 eV, 2.21 eV, 1.93 eV for 1% Ba-doped Bi12GeO20, 2% Mg-doped Bi12GeO20 and 1% Ba, Mg-codoped Bi12GeO20 (Ba
:
Mg = 0.75
:
0.25), which is accordance with the density functional theory (DFT) calculation results in ref. 14. These results demonstrate that the alkaline earth metals modify the electronic structure and crystallite phase. As a result, the novel synthetic photocatalysts were competent to degrade organic contaminants effectively in the irradiation of visible light.
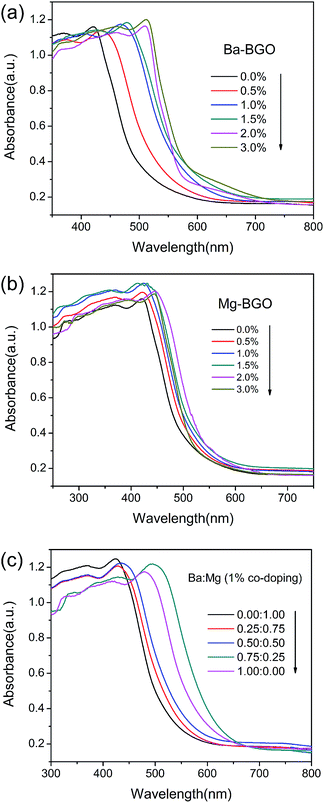 |
| Fig. 4 UV-vis diffuse reflectance spectra of the as-synthesized products: (a) 0.0–3% Ba-doped BGO; (b) 0.0–3% Mg-doped BGO (c) 1% (Ba,Mg)-codoped BGO. | |
3.1.4. PL analysis. Photoluminescence (PL) spectra have been widely used to evaluate the efficiency of charge carrier trapping and understand the fate of electron–hole pairs. The higher the PL intensity is, the higher the recombination rate of photogenerated electron–hole is. The PL emission spectra of pure Bi12GeO20, 1% Ba-doped Bi12GeO20, 2% Mg-doped Bi12GeO20 and 1% (Ba,Mg)-codoped Bi12GeO20 were shown in Fig. 5. Obviously, the PL intensity of doped Bi12GeO20 was weaker than that of pure Bi12GeO20, implying the recombination of photogenerated electron–hole was inhibited effectively. Among all the samples, the co-doped Bi12GeO20 displayed the weakest PL intensity, indicating the high separation effect of photogenerated electron–hole pairs.
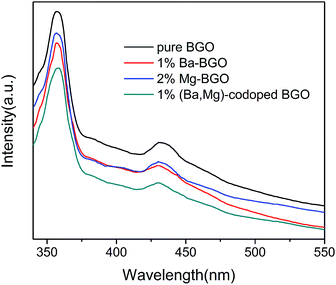 |
| Fig. 5 Photoluminescence spectra of pure BGO, 1% Ba-BGO, 2% Mg-BGO and 1% (Ba,Mg)-codoped BGO. | |
3.1.5. XPS analysis. Elemental composition and chemical states of the sample were explored by using XPS (Fig. 6). The typical full spectra of the 1% co-doped Bi12GeO20 with an Ba
:
Mg ratio of 0.75
:
0.25 confirmed the existence of Ba, Mg, Bi, Ge, C and O elements (Fig. 6a). Carbon can be attributed to adventitious hydrocarbon from the XPS device itself. The high-resolution XPS spectrum of Bi 4f region is presented in Fig. 6b. The spectra exhibited two peaks. The binding energy of 158.1 eV corresponds to Bi 4f7, and the other at 163.4 eV is related to Bi 4f5 chemical state, indicating bismuth in the sample primarily exists in +3 valence state. In Fig. 6c, the peak at 29.1 eV was attributed to Ge 3d binding energy, presenting the +4 valence state of germanium in the sample. The peak observed at 25.9 eV can be ascribed to Bi 5d, which is in close agreement with the previous reports.9 There were three different kinds of peaks for O 1s in the high-resolution XPS spectrum (Fig. 6d). The peaks appeared at 529.1 eV and 530.3 eV can be assigned to the binding energies of Ge–O, Bi–O, and the higher binding energy peak at 531.8 eV can be attributed to the surface hydroxyl groups.11 In addition, Fig. 6e shows the XPS spectra of Ba 3d3 and Ba 3d5 with the peaks at 797.1 and 782.4 eV. Barium existed in the +2 oxidation state. Fig. 6f displays the XPS spectrum of Mg 2p for the co-doped system. The Mg 2p peak is centered at 50.8 eV, which is characteristic of Mg2+. The low doping concentration may take responsibility for the weak signals.21,22 The results indicate the existence of Ba and Mg in Bi12GeO20 crystals, which is in good agreement with the conclusion from the XRD analysis.
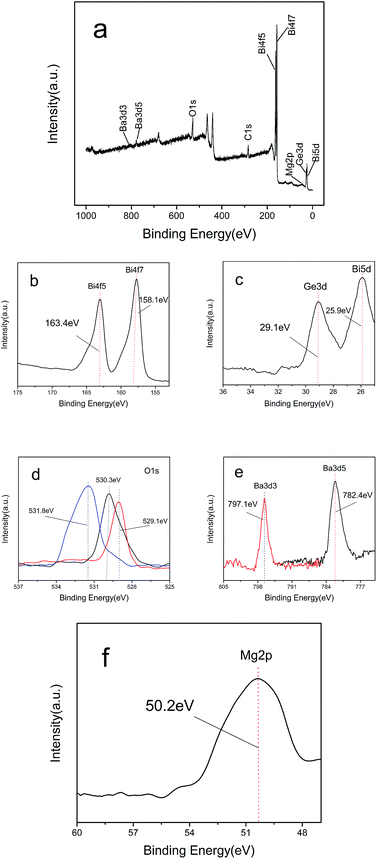 |
| Fig. 6 XPS spectra of (a) survey scan of 1% (Ba,Mg)-codoped BGO (Ba : Mg = 0.75 : 0.25); (b) Bi 4f; (c) Ge 3d and Bi 5d; (d) O 1s; (e) Ba 3d and (f) Mg 2p. | |
3.2. Photocatalytic activity
The photocatalytic activity of the as-prepared samples was investigated by the photodegradation of Rhodamine B (RhB) under visible light irradiation at the room temperature. It is worth noting that there was no measurable reduction in the concentration of RhB under dark condition. The photocatalytic performance of Ba-doped and Mg-doped Bi12GeO20 with different doping content was presented in Fig. 7. It can be seen that doped Bi12GeO20 had higher photoactivity than pure Bi12GeO20. The results can be explained as follows: firstly, low-valence cation Ba2+ and Mg2+ enter the crystal lattice of Bi12GeO20 and substitute Ge4+, resulting in the formation of compensating population of oxygen vacancies, which is favourable to the oxygen diffusion.23–25 The oxygen vacancies act as electron traps to suppress the recombination of photoexcited electrons and holes. On the other hand, the incorporation of metal ion introduces impurity levels in the band gap, making it possible for low-energy photons to generate photogenerated electrons and holes.21 The narrowed band gap as shown in DRS played an important role in the enhancement of photoactivity as well. With the increasing doping concentration the photocatalytic activity of the single-doped Bi12GeO20 increased at first and then decreased. The best photocatalytic activities were achieved when doping amount was 1% and 2% for Ba/BGO and Mg/BGO. A small amount of dopants can effectively separate electron–hole pairs and enhanced the photocatalytic activity. However, when the doping amount is too high, excessive doped ions cannot effectively enter into Bi12GeO20 crystal lattice, but accumulated at the crystal surface.26 The accumulation reduced the active sites of Bi12GeO20 and doping ions may become recombination centre of electrons and holes,27 hence reducing the catalytic activity.
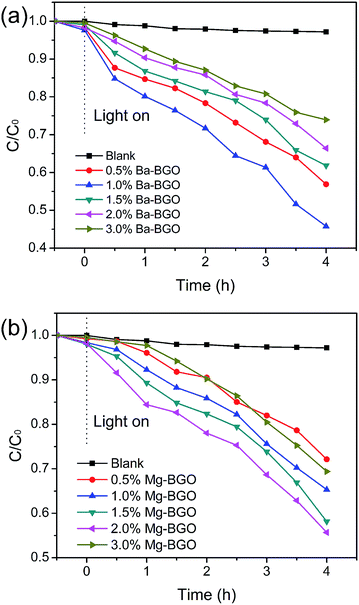 |
| Fig. 7 Photocatalytic degradation of RhB under visible light in presence of (a) Ba-doped and (b) Mg-doped BGO. | |
The degradation rate of RhB over co-doped Bi12GeO20 was shown in Fig. 8a. Obviously, the 1% (Ba,Mg)-codoped Bi12GeO20 with 0.75
:
0.25 Ba
:
Mg exhibited the highest photocatalytic activity among all the co-doped Bi12GeO20. Fig. 8b shows the UV-vis spectra changes of RhB solution in the presence of 1% (Ba,Mg)-codoped Bi12GeO20 (Ba
:
Mg = 0.75
:
0.25). The degradation rate of RhB was very fast in the beginning and then slowed down. The main absorption band showed blue shift, implying that the mainly degradation process of RhB dye is the removal of ethyl groups rather than the decomposition of chromophoric groups.28 Fig. 8c presents the comparison of photodegradation efficiency of RhB over different catalysts. Co-doped Bi12GeO20 have far higher photocatalytic activities than pure and single-doped Bi12GeO20. Only 29%, 54%, 44% of RhB in the solution was photodegraded by pure Bi12GeO20, 1% Ba-doped Bi12GeO20 and 2% Mg-doped Bi12GeO20 after visible light irradiation for 4 h. The concentration of the RhB solution was decreased by 89% when the (Ba,Mg)-codoped Bi12GeO20 was used as the photocatalysts, demonstrating the existence of cooperative effect of barium and magnesium codoping. The enhanced photocatalytic activity can be attributed to the high specific surface area (Table 1) and the narrowed band gap. Compared with that of 2% Mg-doped Bi12GeO20, 1% Ba-doped Bi12GeO20 exhibited higher photocatalytic degradation efficiency due to the lager ionic radius of Ba2+ (r = 0.135 nm) than that of Mg2+ (0.072 nm). Due to the much larger ionic radius of Ba2+, the substitution of Ge4+ (r = 0.053 nm) with Ba2+ has a bigger impact on the lattice structure and causes more oxygen vacancies,29–31 thereby greatly restraining the recombination of photogenerated electrons and holes.
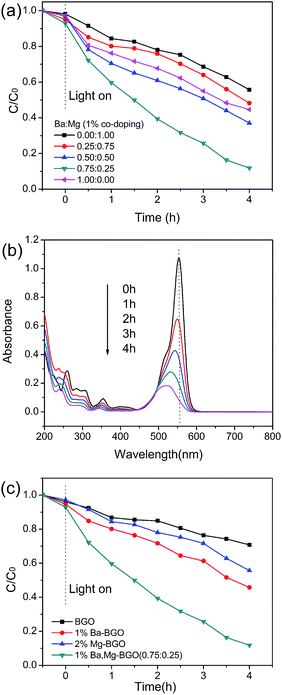 |
| Fig. 8 (a) Photocatalytic degradation of RhB under visible light by 1% (Ba,Mg)-codoped BGO; (b) temporal evolution of the absorption spectral changes during the degradation of RhB by 1% co-doped BGO with 0.75 : 0.25 Ba : Mg. (c) Comparison of photocatalytic degradation of RhB under visible light by pure BGO; 1% Ba-BGO; 2% Mg-BGO; 1% (Ba,Mg)-codoped BGO (Ba : Mg = 0.75 : 0.25). | |
To quantitatively understand the photocatalytic reaction kinetic of RhB, the apparent first order kinetic equation was applied to analyze the experimental date, as expressed by the following equation:
|
 | (4) |
where
C0 and
C (mg L
−1) are the RhB concentration in solution at time 0 and
t respectively, and
k is the apparent first order rate constant (min
−1). The rate constants of each sample in
Fig. 8c were 0.0886, 0.1838, 0.1308, 0.4979 for pure Bi
12GeO
20, 1% Ba-doped Bi
12GeO
20, 2% Mg-doped Bi
12GeO
20 and 1% Ba/Mg-BGO (Ba
![[thin space (1/6-em)]](https://www.rsc.org/images/entities/char_2009.gif)
:
![[thin space (1/6-em)]](https://www.rsc.org/images/entities/char_2009.gif)
Mg = 0.75
![[thin space (1/6-em)]](https://www.rsc.org/images/entities/char_2009.gif)
:
![[thin space (1/6-em)]](https://www.rsc.org/images/entities/char_2009.gif)
0.25) respectively, and shown in
Table 1. Obviously, the results were corresponding to the photocatalytic degradation effect: the co-doped Bi
12GeO
20 have higher photodegradation rate than others.
3.3. Visible light photodegradation mechanism
Hydroxyl radical (˙OH), superoxide radical (˙O2−) and photogenerated holes (h+) play important roles in photocatalytic degradation of organic contaminants. Various radical scavengers were used to explore the photodegradation mechanism of RhB solution with (Ba,Mg)-codoped BGO as the photocatalyst. Benzoquinone (BQ) was applied as the scavenger of superoxide radical, and isopropanol (IPA) was adopted as the scavenger of hydroxyl radical, and triethanolamine (TEOA) was employed as the scavenger of holes. As shown in Fig. 9, compared with no scavenger under otherwise identical condition, the addition of TEOA had dramatically inhibited the photocatalysis, indicating that photogenerated holes played a dominant role in the degradation of RhB. The presence of IPA brought certain reduction in the degradation efficiency of RhB, hence ˙OH radical can be considered as the secondary active species.29 Meanwhile, the removing efficiency of RhB showed slight drop after BQ was added into the reaction system, which indicated that ˙O2− radical made a minor contribution on photocatalytic process.
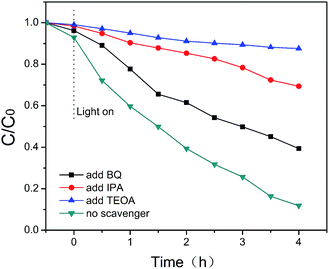 |
| Fig. 9 The effects of different scavengers on the degradation of RhB over 1% Ba/Mg-BGO (Ba : Mg = 0.75 : 0.25) under visible light irradiation. | |
According to the aforementioned experimental results, a possible reaction mechanism for photocatalytic process over the (Ba,Mg)-codoped Bi12GeO20 was proposed. The substitution of Ge4+ with Ba2+and Mg2+ leads to the change of lattice structure and the increase of oxygen vacancy which bind excited electrons. Thus, the remaining free holes directly react with the dye. The hydroxyl is absorbed and oxidized into hydroxyl radical by the holes. Meanwhile, the negative charge e− react with the oxygen absorbed on the surface of the photocatalysts to generate ˙O2− radical, which can directly react with the dye.32 Therefore, the photocatalytic properties of the (Ba,Mg)-codoped Bi12GeO20 are greatly improved and the photocatalytic procedure is presented as follows:
|
Ba/Mg-BGO + hν → Ba/Mg-BGO (e− + h+)
| (5) |
|
h+ + RhB → degradation products
| (6) |
|
˙OH + RhB → degradation products
| (9) |
|
˙O2− + RhB → degradation products
| (11) |
3.4. Stability evaluation
To study the stability and repeatability of the sample, cycling experiments over (Ba,Mg)-codoped Bi12GeO20 were implemented repeatedly in three times (Fig. 10). It exhibited the rapid degradation and high photoactivity under repeated application, indicating that the deactivation of the catalyst nearly did not happen. The result demonstrated that (Ba,Mg)-codoped Bi12GeO20 was a stable and efficient photocatalyst under such experimental reaction conditions.
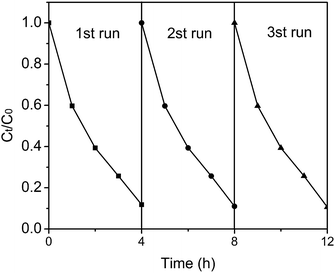 |
| Fig. 10 The cycling runs of the degradation of RhB over co-doped BGO under visible light illumination. | |
4. Conclusion
In summary, (Ba,Mg)-codoped Bi12GeO20 had been successfully synthesized by the one-step hydrothermal method. Various characterization techniques were adopted to study the properties of the as-prepared photocatalysts. It was confirmed that barium and magnesium entered into the Bi12GeO20 crystal lattice. The band gap of co-doped Bi12GeO20 was remarkably narrowed compared with pure and single-doped Bi12GeO20. Obviously, 1% co-doped Bi12GeO20 containing 0.75
:
0.25 Ba
:
Mg displayed highest photocatalytic activity for the degradation of RhB solution under visible light irradiation. The enhanced photocatalytic performance can be ascribed to the effective separation of photogenerated electron–hole pairs and the narrowed band gap. Furthermore, the free radical experiments demonstrated that the photogenerated holes were the dominate factors responsible for the photocatalytic degradation, meanwhile hydroxyl radical and superoxide radical played secondary and minor roles respectively. This paper provided experimental and theoretical basis for the further practical application of the Bi12GeO20 photocatalysts.
Acknowledgements
This work was supported by Guangdong Water and Air Pollution Control Key Laboratory (2001 A060901002) open funded project.
Notes and references
- A. Fujishima and K. Honda, Nature, 1972, 238, 37–38 CrossRef CAS PubMed
. - S. Wu, J. Fang, X. Xu, Z. Liu, X. Zhu and W. Xu, Photochem. Photobiol., 2012, 88, 120–1210 CrossRef PubMed
. - S. Wu, J. Fang, X. Hong, K. S. Hui and Y. Chen, Dalton Trans., 2014, 43, 2611–2619 RSC
. - Y. Chen, X. Xu, J. Fang, G. Zhou, Z. Liu, S. Wu, W. Xu, J. Chu and X. Zhu, Sci. World J., 2014, 2014, 647040 Search PubMed
. - Z. Zhang, W. Wang, M. Shang and W. Yin, J. Hazard. Mater., 2010, 177, 1013–1018 CrossRef CAS PubMed
. - W. Xu, G. Zhou, J. Fang, Z. Liu, Y. Chen and C. Cen, Int. J. Photoenergy, 2013, 2013, 1–9 Search PubMed
. - W. Xu, Z. Liu, J. Fang, G. Zhou, X. Hong, S. Wu, X. Zhu, Y. Chen and C. Cen, Int. J. Photoenergy, 2013, 2013, 1–7 Search PubMed
. - C. He and M. Gu, Scr. Mater., 2006, 54, 1221–1225 CrossRef CAS
. - Z. Wan and G. Zhang, Sci. Rep., 2014, 4, 6298 CrossRef CAS PubMed
. - D. Hou, X. Hu, Y. Wen, B. Shan, P. Hu, X. Xiong, Y. Qiao and Y. Huang, Phys. Chem. Chem. Phys., 2013, 15, 20698–20705 RSC
. - Q. Han, J. Zhang, X. Wang and J. Zhu, J. Mater. Chem. A, 2015, 3, 7413–7421 CAS
. - X. Zhu, J. Zhang and F. Chen, Appl. Catal., B, 2011, 102, 316–322 CrossRef CAS
. - W. Feng Yao, H. Wang, X. Hong Xu, Y. Zhang, X. Na Yang, S. Xia Shang, Y. Hui Liu, J. Tao Zhou and M. Wang, J. Mol. Catal. A: Chem., 2003, 202, 305–311 CrossRef
. - J. Lu, Y. Dai, Y. Zhu and B. Huang, ChemCatChem, 2011, 3, 378–385 CrossRef CAS
. - G. Jiang, X. Wang, Z. Wei, X. Li, X. Xi, R. Hu, B. Tang, R. Wang, S. Wang, T. Wang and W. Chen, J. Mater. Chem. A, 2013, 1, 2406 CAS
. - D. Dolat, N. Quici, E. Kusiak-Nejman, A. W. Morawski and G. Li Puma, Appl. Catal., B, 2012, 115–116, 81–89 CrossRef CAS
. - X. Yang, L. Xu, X. Yu and Y. Guo, Catal. Commun., 2008, 9, 1224–1229 CrossRef CAS
. - R. Yuan, B. Zhou, D. Hua and C. Shi, J. Hazard. Mater., 2013, 262, 527–538 CrossRef CAS PubMed
. - Z. Jiao, T. Chen, J. Xiong, T. Wang, G. Lu, J. Ye and Y. Bi, Sci. Rep., 2013, 3, 2720 Search PubMed
. - Y. Chen, J. Fang, S. Lu, W. Xu, Z. Liu, X. Xu and Z. Fang, J. Chem. Technol. Biotechnol., 2015, 90, 947–954 CrossRef CAS
. - M. Nasir, S. Bagwasi, Y. Jiao, F. Chen, B. Tian and J. Zhang, Chem. Eng. J., 2014, 236, 388–397 CrossRef CAS
. - B. Benalioua, M. Mansour, A. Bentouami, B. Boury and H. Elandaloussi el, J. Hazard. Mater., 2015, 288, 158–167 CrossRef CAS PubMed
. - S. Wu, J. Fang, W. Xu and C. Cen, J. Chem. Technol. Biotechnol., 2013, 88, 1828–1835 CrossRef CAS
. - J. Huo, L. Fang, Y. Lei, G. Zeng and H. Zeng, J. Mater. Chem. A, 2014, 2, 11040 CAS
. - J. Cao, B. Xu, H. Lin, B. Luo and S. Chen, Chem. Eng. J., 2012, 185–186, 91–99 CrossRef CAS
. - C. Yin, S. Zhu, Z. Chen, W. Zhang, J. Gu and D. Zhang, J. Mater. Chem. A, 2013, 1, 8367 CAS
. - H. Shi, H. Tan, W.-b. Zhu, Z. Sun, Y. Ma and E. Wang, J. Mater. Chem. A, 2015, 3, 6586–6591 CAS
. - W. Xu, J. Fang, Y. Chen, S. Lu, G. Zhou, X. Zhu and Z. Fang, Mater. Chem. Phys., 2015, 154, 30–37 CrossRef CAS
. - Y. K. Lai, J. Y. Huang, H. F. Zhang, V. P. Subramaniam, Y. X. Tang, D. G. Gong, L. Sundar, L. Sun, Z. Chen and C. J. Lin, J. Hazard. Mater., 2010, 184, 855–863 CrossRef CAS PubMed
. - Y. Chen, J. Fang, S. Lu, Y. Wu, D. Chen, L. Huang, W. Xu, X. Zhu and Z. Fang, Mater. Res. Bull., 2015, 64, 97–105 CrossRef CAS
. - Y. Yang, G. Zhang and W. Xu, J. Colloid Interface Sci., 2012, 376, 217–223 CrossRef CAS PubMed
. - Y. Zhang, W. Zhu, X. Cui, W. Yao and T. Duan, CrystEngComm, 2015, 17, 8368–8376 RSC
.
|
This journal is © The Royal Society of Chemistry 2016 |
Click here to see how this site uses Cookies. View our privacy policy here.