DOI:
10.1039/C6QI00254D
(Research Article)
Inorg. Chem. Front., 2016,
3, 1381-1387
Intense blue emission and a reversible hypsochromic shift of luminescence caused by grinding based on silver(I) complexes†
Received
16th July 2016
, Accepted 23rd August 2016
First published on 26th August 2016
Abstract
Silver(I) complexes bearing N-heterocyclic carbene and diphenylphosphinobenzene ligands, [Ag(IPr)(dppbz)]BPh4 (1, IPr = bis(2,6-diisopropylphenyl)imidazole-2-ylidene, dppbz = 1,2-bis(diphenylphosphino)benzene), [Ag(IPr)(dfpbz)]BPh4 (2, dfpbz = 1,2-bis[di(4-fluorophenyl)phosphino]benzene), and [Ag(IPr)(dtbpbz)]BPh4 (3, dtbpbz = 1,2-bis[bis[3,5-di(tert-butyl)phenyl]phosphino]benzene), were newly synthesized and characterized. These complexes, especially 3, exhibit intense blue emission in the solid state. The maximum wavelength and lifetime of the photoluminescence of 3 are 440 nm and 14 μs, respectively. The quantum yield of the photoluminescence of 3 in the solid state is 0.5, which is one of the highest values among blue-emissive silver(I) complexes ever reported. Introduction of bulky tert-butyl groups at 3- and 5-positions of the phenyl groups in the diphosphine ligand plays a key role in both the blue-shift and the enhancement of the photoluminescence. Additionally, the reversible hypsochromic shift of the long-lived emission caused by the grinding process is observed for one of the complexes.
Introduction
Metal complexes bearing N-heterocyclic carbene (NHC) ligands, such as 1,3-bis(2,6-diisopropylphenyl)imidazole-2-ylidene (IPr), have been extensively studied as catalysts.1–3 In recent years, photoluminescence related to the long-lived triplet state of NHC complexes has attracted considerable attention.4–17 Copper(I) complexes bearing IPr and 1,2-bis(diphenylphosphino)benzene (dppbz) ligands, [Cu(IPr)(dppbz)]+, have been known to exhibit long-lived intense blue luminescence.18 The bis(dppbz) silver(I) complex, [Ag(dppbz)2]+, is also known to be luminescent.19 Silver(I) complexes bearing NHC ligands are important as the starting materials of many kinds of metal NHC complexes,20 however, the number of reports for highly luminescent silver(I) complexes bearing the NHC ligand with a microsecond lifetime is very limited. It should also be noted that materials emitting strong blue light are required for manufacturing OLED devices.21
Luminescence mechanochromism is often based on fluorescent dyes such as organic compounds, macromolecules, and zinc complexes.22,23 Recently some silver, gold and platinum complexes have been reported to show luminescence mechanochromism related to the triplet state on grinding the solid samples.24 In many cases, the bathochromic shift of the emission by a mechanical process has been reported. The hypsochromic shift (20 nm) of emission spectra on grinding was only reported for a copper coordination polymer.25
We examined detailed photophysical parameters, quantum yields and lifetimes of the photoluminescence of some silver(I) complexes in the solid state, and found that these complexes show long-lived intense blue emission as well as the reversible hypsochromic shift on grinding. We describe herein the detailed photophysics of [Ag(IPr)(dppbz)]BPh4 (1), [Ag(IPr)(dfpbz)]BPh4 (2, dfpbz = 1,2-bis[di(4-fluorophenyl)phosphino]benzene) and a highly blue-emissive silver(I) complex bearing IPr and 1,2-bis[bis(3,5-di-tert-butyl-phenyl)phosphino]benzene (dtbpbz) ligands, [Ag(IPr)(dtbpbz)]BPh4 (3) (Scheme 1).
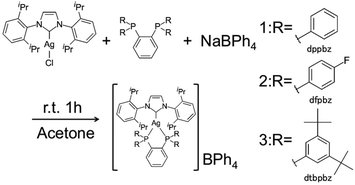 |
| Scheme 1 [Ag(IPr)(dppbz)]BPh4 (1), [Ag(IPr)(dfpbz)]BPh4 (2), and [Ag(IPr)(dtbpbz)]BPh4 (3). | |
Results and discussion
All complexes were newly synthesized by the reaction of diphosphines (dppbz, dfpbz, and dtbpbz), NaBPh4 and chloro[1,3-bis(2,6-diisopropylphenyl)imidazole-2-ylidene]silver ([AgCl(IPr)]) in acetone and obtained as white powders. The compounds were characterized by 1H NMR, elemental analysis, and single-crystal X-ray structural analysis. Crystal structures of 1 and 2 are shown in Fig. 1. The structures of the heteroleptic mononuclear silver(I) complexes bearing a N-heterocyclic carbene ligand and a bidentate diphosphine ligand with trigonal planar coordination geometry were confirmed for both 1 and 2. Furthermore, the sums of bond angles around the silver atom are almost 360° for both 1 and 2, therefore the coordination geometry of both the complexes shows high planarity. The P–Ag–P angles of 1 and 2 are 78.61(3) and 79.29(5), respectively. The P–Ag–P angles are similar to that of [Ag(dppbz)2]+ (79.99(2)).19a In addition, the bond lengths of Ag–C of 1 and 2 are 2.122(3) and 2.134(5) Å, respectively, which are close to the reported range of the Ag–C length (2.1295(18) Å) in the literature.26 In addition, the space group of 1 is P41, which is a chiral space group, and 1 indicated a helical structure formed by the intermolecular interaction between cations and the counter anions (Fig. S1†). Considering the fact that the van der Waals radius of a carbon atom is 1.7 Å, there should be weak interactions between a phenyl group of one of the BPh4− anions and the imidazole groups of the two NHC ligands and between the phenyl groups of the dppbz ligands on the different complex cations. However the interaction should be weak because the interacting aromatic groups are not parallelly oriented.
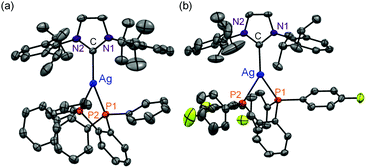 |
| Fig. 1 The crystal structures of the complex cations of 1 (left) and 2 (right). A complex cation, hydrogens, counter ions, and solvent molecules are omitted for clarity. Important bond length (Å) and angles (°) for 1: Ag–C, 2.144(3); Ag–P1, 2.4868(9); Ag1–P2, 2.5344(9); C–Ag–P1, 145.37(9); C–Ag–P2, 135.97(9); P1–Ag–P2, 78.61(3). For 2: Ag–C, 2.134(5); Ag–P1, 2.4969(13); Ag–P2, 2.5108(14); C–Ag–P1, 141.77(15); C–Ag–P2, 138.91(15); P1–Ag1–P2, 79.29(5). | |
The 1H NMR spectra of the complexes showed a coordinated NHC carbene ligand and a diphosphine ligand with a 1
:
1 molar ratio. It is often observed that 31P NMR spectra of silver complexes show a broad signal due to the rapid exchange equilibrium.27 However, the 31P{1H} NMR spectra of 1, 2 and 3 in CD2Cl2 show clearly split signals, by 1JP–Ag(107) and 1JP–Ag(109) couplings. In addition, the 1H NMR spectra of the three complexes remained unchanged for 3 days in CD2Cl2 stored in the dark. The result shows that the complexes practically retain the three-coordinate geometry in the solution for at least several days (Fig. S2–S10†).
Absorption spectra of all the complexes show intense absorption around 260–300 nm due to the ligand centered transition (Fig. S11a and b†). All complexes have a weak shoulder at around 330 nm. The molar extinction coefficients (ε) at the shoulder bands are 4.6 × 103 M−1 cm−1. The wavelengths at the emission maximum of 1 and 3 in the degassed dichloromethane at room temperature are 473 nm and 465 nm (Fig. 2a and Table 1), respectively. 3 shows stronger emission than 1. 2 exhibits weak emission, but the luminescence is similar to that of the free ligand (dfpbz: 384 nm, Fig. S12†). So we concluded that the luminescence observed in the solution of 2 mainly comes from trace amounts of the dissociated free ligand. It should be noted that the emissions of other free ligands, dppbz or dtbpbz, are very weak in the degassed dichloromethane solution and are completely different from those of 1 and 3. In CH2Cl2, the lifetimes of 1 and 3 are in the microsecond range (Fig. S13a†). The longer lifetime and larger quantum efficiency of 3 in CH2Cl2 than 1 are due to the smaller knr value of 3 (Table S1†). This may be caused by the small structural change at the excited state of 3 and effective shielding from the solvents by the bulky diphosphine, dtbpbz. Some silver(I) complexes with diphosphine ligands are known to emit phosphorescence: [Ag(dppbz)2]+ (τ = 28 μs), [Ag(dppe)2]+ (τ = 12 μs).19a Emissions of 1, 2 and 3 are considered as phosphorescence similarly. The tetrahedral complex, [Ag(dppbz)2]+,19a indicates a remarkable large Stokes-shift of the emission in solution by the flatting distortion, however, trigonal planar complexes, 1 and 3, showed a smaller Stokes-shift of the emission than the tetrahedral complex, because the structural change of the trigonal planar complexes is more reduced than that of the tetrahedron complex.28 In addition, the emission intensity of [Ag(dppbz)2]+ in degassed dichloromethane decreased soon by UV irradiation.19b1 and 3, on the other hand, in the degassed dichloromethane were irradiated by Xe lamps, and the emission spectra were unchanged (Fig. S14a and b†). 3 is more photochemically stable, because 3 has the bulky substituent, tert-butyl, which should prevent the centred metal from coordination of the solvent molecules.
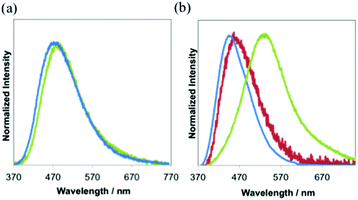 |
| Fig. 2 (a) Emission spectra of 1 (green) and 3 (blue) in degassed dichloromethane at room temperature (uncorrected). (b) Emission spectra of 1 (green), 2 (red) and 3 (blue) in the solid state (uncorrected). | |
Table 1 Photophysical properties of 1, 2, and 3 in the degassed CH2Cl2 and in the solid state
Complexes |
λ
em, solution a/nm |
τ
solution a/μs |
Φ
solution a |
λ
em, solid b/nm |
λ
em, solid c/nm |
τ
solid b/μs |
τ
solid c/μs |
Φ
solid b |
Φ
solid c |
In degassed CH2Cl2.
As synthesized.
After grinding.
Excited by 350 nm.
Excited by 355 nm.
Excited by 345 nm.
The decay curve of these complexes was fitted with the double-exponential curve (I = a exp(−t/τ1) + b exp(−t/τ2)) and parentheses show aτ1/(aτ1 + bτ2) and bτ2/(aτ1 + bτ2) respectively.
The value of Φ of 1+ has a significant error considering the immediate changes in emission spectra in degassed CH2Cl2 during measurements.
|
1
|
473d |
1.1 |
0.018d,h |
523d |
494e |
36 (88%), 7.8 (12%)g |
12.2 (72%), 4.0 (28%)g |
0.36d |
0.16d |
2
|
Not detected |
Not measured |
Not measured |
455d |
455f |
9.8 (80%), 2.5 (20%)g |
5.8 (87%), 0.82(13%)g |
0.074d |
0.053d |
3
|
465e |
6.3 |
0.092e |
440e |
440d |
14 (96%), 1.4 (4%)g |
9.2(37%), 3.3(63%)g |
0.46e |
Not measured |
In the solid state, both 2 and 3 exhibit blue luminescence with peak maxima at 455 nm and 440 nm (Table 1 and Fig. 2b, S15†). On the other hand, 1 shows blue-green luminescence with λem of 523 nm. Intermolecular interactions between the cation and the counter anion should lower the energy gap in the crystal. The CIE coordinates of 1, 2 and 3 are (0.29, 0.43), (0.16, 0.16) and (0.15, 0.10), respectively (Fig. S15†). The quantum yield, Φ, of the luminescence in the solid state of 3 at room temperature is 0.46. The value is slightly larger than that of 1 (0.36) under the same conditions. The emission decay curves of all complexes in the solid state are the best fit to a double-exponential curve (Fig. S13b–g†). The longer components of the lifetimes of the emission, τ, of 1, 2 and 3 are 36 (88%) μs, 9.8 (80%) μs and 14 (96%) μs, respectively. These values are characteristic of the triplet excited states.
The emission of the as-synthesized sample of 1 shows a striking hypsochromic shift by 30 nm on grinding the sample in a mortar, and the blue-green emission was restored by adding a portion of diethylether followed by drying under air, or heating the sample at 100 °C for 40 min (Fig. 3a–e). As described above, 1 forms the helical array of the cations and anions in the crystal made by intermolecular interaction between the imidazole moiety in the NHC ligand and the phenyl groups of the BPh4− anion and between the dppbz ligands. The result that the interaction is very weak in the crystal may be the reason why the interactions were broken on grinding. The fact that the emission band of G1 is still more red shifted than that of 1 in CH2Cl2 shows that some interactions were still working in the amorphous form (see later) of 1. The CIE coordinate of the ground sample of 1, G1, is (0.22, 0.32), which is obviously blue compared to that of the original sample of 1 (Fig. S15†). The longer component of the lifetimes of the emission (12.2 μs) and the quantum yield (0.16) were smaller than those of the original sample. The 1H NMR observed in CD2Cl2 of G1 was the same as that of the original sample. The powder X-ray diffraction (PXRD) signals indicated that 1 becomes amorphous on grinding, and adding some drops of diethylether to G1 restores the PXRD signal pattern of 1. The hypsochromic shift of 1 on grinding can be caused by the breaking of the intermolecular interactions between the ligands and the counter anions during the mechanical process (Fig. S1†).25,29,30 In contrast, 2 and 3 show no change in the wavelength of the emission maximum on grinding, although the lifetimes of 2 and 3 shortened.
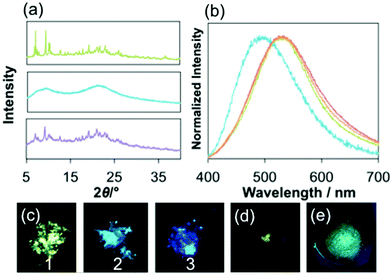 |
| Fig. 3 (a) The PXRD pattern of as synthesised 1 (green, upper), after grinding (blue-green, middle), after adding a portion of ether (purple, lower). (b) Emission spectrum of 1 as synthesised (green), after grinding (blue-green), after addition of a portion of diethyl ether (orange), after heating the ground sample at 100 °C (red) (excited by 355 nm, uncorrected). (c) Pictures of 1 (left), 2 (centre) and 3 (right) excited by a UV lamp. (d) Picture of as-synthesized powder of 1 on an agate mortar under UV light. (e) Picture of ground powder of 1 on an agate mortar under UV light. | |
DFT calculations were performed to discuss the photophysics of the complexes, 1–3, (Table 2, Fig. 4 and S16†). The energies and the components of the important orbitals for the optimized structure are described in Table 2. The important bond lengths and angles of the singlet-optimized structures of 1 and 2 are similar to those of the crystal structures (Table S2†). In these complexes, the HOMOs are made of the atomic orbitals of the phosphorus atoms, the phenyl groups of the diphosphine ligand and the silver atoms. The unoccupied orbitals (LUMO, LUMO+1 and LUMO+2) mainly consist of π* orbitals of the diphosphine ligand. The characteristics of these orbitals of 1–3 are similar but the LUMO+2 and LUMO+3 of 3 are reversed from those of 1 and 2.
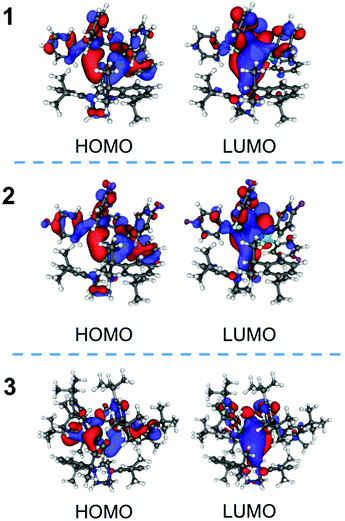 |
| Fig. 4 Calculated HOMO and LUMO of 1, 2 and 3. | |
Table 2 Result of DFT calculations based on the singlet optimized structures
|
K–S orbital |
Energy/eV |
Aga |
PPb |
Phc |
IPrd |
Component moiety of silver (%).
Component moiety of two phosphorus atoms.
Component moiety of the diphosphine ligand without two phosphorus atoms.
Component moiety of IPr.
|
1
|
LUMO+4 |
−2.56 |
0 |
7 |
82 |
11 |
LUMO+3 |
−2.68 |
17 |
1 |
47 |
35 |
LUMO+2 |
−2.87 |
4 |
16 |
75 |
5 |
LUMO+1 |
−3.17 |
3 |
13 |
83 |
1 |
LUMO |
−3.19 |
13 |
17 |
60 |
9 |
HOMO |
−8.01 |
18 |
40 |
38 |
4 |
HOMO−1 |
−8.99 |
4 |
8 |
62 |
26 |
2
|
LUMO+4 |
−2.70 |
1 |
6 |
83 |
11 |
LUMO+3 |
−2.93 |
16 |
4 |
59 |
21 |
LUMO+2 |
−3.04 |
3 |
14 |
81 |
3 |
LUMO+1 |
−3.34 |
8 |
17 |
71 |
3 |
LUMO |
−3.39 |
9 |
14 |
70 |
7 |
HOMO |
−8.19 |
17 |
37 |
42 |
4 |
HOMO−1 |
−9.06 |
4 |
8 |
85 |
3 |
3
|
LUMO+4 |
−2.30 |
4 |
5 |
78 |
13 |
LUMO+3 |
−2.49 |
10 |
8 |
72 |
9 |
LUMO+2 |
−2.52 |
12 |
12 |
52 |
24 |
LUMO+1 |
−2.91 |
3 |
9 |
85 |
3 |
LUMO |
−2.99 |
20 |
18 |
51 |
12 |
HOMO |
−7.72 |
16 |
37 |
45 |
2 |
HOMO−1 |
−8.52 |
0 |
1 |
98 |
1 |
The TDDFT calculation for 1 indicates that the largest component of the transition from the ground (S0) to the singlet lowest-lying excited (S1) state (S0 → S1) is HOMO → LUMO (Table S3†). The character of the transition of 1 was the mainly intraligand transition of the dppbz moiety. The characters of 2 and 3 were similar to that of 1. The largest component among the transitions from the ground to the triplet excited (T1) states (S0 → T1) of 1 is HOMO → LUMO, which is similar in nature to that of the S0 → S1 transition (Table S3†). The results of TDDFT calculations of 1–3 indicated that the emission properties of the complexes are related to the orbitals of the diphosphine ligands with a small contribution from the silver atoms. The results suggested that the emission of 1–3 can be similar to [Ag(dppbz)2]+. However, the wavelength of the emission of [Ag(dppbz)2]+ in degassed solution is long (670 nm–680 nm), because a large flattening distortion of [Ag(dppbz)2]+ in the excited state was proposed.19 On the other hand, our complexes, 1–3, have a three-coordinate geometry, which reduces the distortion in the excited state and leads to blue photoluminescence.
Conclusions
Silver(I) complexes bearing IPr and diphosphine ligands, 1, 2 and 3 with long-lived intense blue emission were newly synthesized. The tert-butyl group in the dtbpbz ligand has considerable effects on stabilizing the complex in the solution state and increasing both the quantum yield and the lifetime. The powder of 1 exhibits a reversible hypsochromic shift of the emission upon grinding, and the original emission is restored upon heating or adding diethylether to the sample. The emission spectrum in the solid state shows a hypsochromic shift on grinding, although many other mechanochromic complexes with microsecond lifetime show a bathochromic shift on grinding. Our research is important for the colouring matter of a high efficiency device.
Experimental
Materials and methods
1,2-Bis(diphenylphosphino)benzene (dppbz) and chloro[1,3-bis(2,6-diisopropylphenyl)imidazol-2-ylidene]silver (AgCl(IPr)) were purchased from Tokyo Chemical Industry CO., Ltd. 1,2-Bis[bis[3,5-di(tert-butyl)phenyl]phosphino]benzene (dtbpbz) was purchased from Wako Pure Chemical Industry CO., Ltd. 1,2-Bis[di(4-fluorophenyl)phosphino]benzene (dfpbz) was prepared by a reported reaction31 of 1,2-bis(dichlorophosphino)benzene and 4-fluorophenylmagnesium bromide solution in THF, both of which were purchased from Sigma-Aldrich. 1H-NMR and 31P-NMR spectra in CD2Cl2 were recorded on a JEOL Delta-500 spectrometer using tetramethylsilane (δ = 0.00 ppm, 1H) as an internal standard,32 and 85% phosphoric acid (δ = 0.00 ppm, 31P) as an external standard. Emission spectra were obtained in a solvent degassed by at least five freeze–pump–thaw cycles using a quartz cell fitted with a Teflon vacuum stopcock. Absorption spectra were recorded on an Agilent 8453 UV-visible spectrometer. Emission spectra and emission lifetimes were collected on a laboratory-made apparatus. Detailed methods were reported in the literature.33 Emission quantum yields in solution were obtained from the sensitivity corrected spectrum using 9,10-diphenylanthracene as a standard (Φ = 0.97 in cyclohexane).34 The quantum yields in the solid state were measured by the absolute method using an integrating sphere. The PXRD pattern was measured on a Rigaku Ultima IV X-ray diffractometer (40 kV, 40 mA).
Synthesis
[Ag(IPr)(dppbz)]BPh4 (1).
Under an argon atmosphere, AgCl(IPr) (105 mg, 0.20 mmol), dppbz (92 mg, 0.21 mmol), and NaBPh4 (68 mg, 0.20 mmol) were added to 5 mL of acetone. The reaction mixture was stirred for 60 min at room temperature. The white solid deposited was filtered off, and the filtrate was evaporated to dryness, then the solid obtained from the filtrate was washed with methanol, and dried in vacuo: a white powder, yield, 151.7 mg (0.12 mmol, 61%). 1H NMR (500 MHz, CD2Cl2) 1H NMR, δ 7.55(m, 2H), 7.41(m, 2H), 7.32–7.25(m, 18H), 7.15(m, 2H), 7.12(t, 10H), 7.02(t, 8H), 6.87(t, 4H), 6.54(m, 8H), 2.64(sep, J = 7.0 Hz, 4H, CH3CHCH3), 1.16(d, J = 7.0 Hz, 12H, CH3CHCH3), 0.93(d, J = 7.0 Hz, 12H, CH3CHCH3) 31P{1H} NMR (202 MHz, CD2Cl2) −4.31 (d, 1JP–Ag(107) 234 Hz), −4.30(d, 1JP–Ag(109) 269 Hz). Anal. Found: C, 76.74; H, 6.36; N, 2.1%. Calcd for 1, C81H80N2P2BAg: C, 77.08; H, 6.39; N, 2.22.
[Ag(IPr)(dfpbz)]BPh4 (2).
2 was synthesized according to a similar procedure to that of 1 by employing AgCl(IPr) (200 mg, 0.38 mmol), dfpbz (176 mg, 0.38 mmol), and NaBPh4 (120 mg, 0.35 mmol) with a modification of the reaction temperature from room temperature to −40 °C. The product is a white powder, yield, 316 mg (0.24 mmol, 77%). 1H NMR (500 MHz, CD2Cl2) δ 7.58(m, 2H), 7.46(m, 2H), 7.34–7.28 (m, 14H), 7.12(m, 2H), 7.02(t, 8H), 6.87(t, 12H), 6.50(m, 8H), 2.64(quin, J = 7.0 Hz, 4H, CH3CHCH3), 1.19(d, J = 7.0 Hz, 12H, CH3CHCH3), 0.95(d, J = 7.0 Hz, 12H, CH3CHCH3) 31P{1H} NMR (202 MHz, CD2Cl2) −6.62 (d, 1JP–Ag(107) = 234 Hz), −6.62 (d, 1JP–Ag(109) = 269 Hz). Anal. Found: C, 72.52; H, 5.72; N, 2.07%. Calcd for 2, C81H76N2P2BF4Ag: C, 72.92; H, 5.74; N, 2.10.
[Ag(IPr)(dtbpbz)]BPh4 (3).
3 was synthesized according to a similar procedure to that of 1 by employing AgCl(IPr) (53.7 mg, 0.10 mmol), dtbpbz (88.1 mg, 0.098 mmol), and NaBPh4 (34.0 mg, 0.099 mmol) with a modification of the reaction temperature from room temperature to −41 °C. The product is a white powder, yield, 91 mg (0.053 mmol, 54%). 1H NMR (500 MHz, CD2Cl2), δ 7.36(m, 6H), 7.30(br, 8H), 7.12(d, 2H), 7.20–7.16(br, 2H), 7.08–7.01(m, 14H), 6.87(m, 4H), 6.62(m, 8H), 2.65(quin, J = 7.0 Hz, 4H, CH3CHCH3), 1.13–1.10(m, 86H), 1.03(d, J = 7.0 Hz, 12H, CH3CHCH3) 31P{1H} NMR (202 MHz, CD2Cl2) δ −2.07(d, 1JP–Ag(107) = 234 Hz), −2.07(d, 1JP–Ag(109) = 269 Hz). Anal. Found: C, 78.66; H, 8.19; N, 1.57. Calcd for 3·acetone, C113H144N2P2BAg·C3H6O: C, 78.76; H, 8.55; N, 1.58.
Crystallography
X-ray crystallographic measurements of 1 and 2 were made on a Rigaku Saturn 70 CCD area detector with graphite-monochromated MoKα radiation. Images were collected and the data were processed by using CrystalClear.35 The structures were solved by a direct methods SIR-92
36 and refined by full matrix least squares procedures (SHELXL-97).37 All calculations were performed by using the Wingx crystallographic software package.38 Crystallographic data have been deposited to the Cambridge Crystallographic Data Centre: CCDC 1472559 for 1 and CCDC 1472558 for 2. Single crystals suitable for the analyses were obtained by slow diffusion of diethylether in acetone solution of 1 and 2, respectively. Crystal data of 1: C81H80AgBN2P2, Mr = 1262.09, colorless crystal, tetragonal, space group P41, a = b = 17.4551(1) Å, c = 44.281(3) Å, α = β = γ = 90°, V = 13
491.4(16) Å3, Z = 8, T = 123 K, Dc = 1.243 g cm−3, μ = 0.392 mm−1, R = 0.0493 for 27
318 reflections with I > 2σ(I), Rw = 0.1048, GOF = 1.063, Flack parameter −0.018(12); crystal data of 2·2CH2Cl2: C83H80AgBCl4F4N2P2, Mr = 1503.91, colorless crystal, triclinic, space group P
, a = 13.9331(13) Å, b = 14.8995(13) Å, c = 19.1156(17) Å, α = 88.373(13)°, β = 85.477(12)°, γ = 71.782(10)°, V = 3757.6(6) Å3, Z = 2, T = 123 K, Dc = 1.329 g cm−3, μ = 0.508 mm−1, R = 0.0935 for 13
834 reflections with I > 2σ(I), Rw = 0.2426, GOF = 1.105.
DFT calculation
Calculations were performed using the Gaussian 03 W and Gaussian 09 software39 with the pbe1pbe40 method using the atomic coordinates determined by X-ray as the initial coordinates in the optimization. TDDFT was used to calculate singlet and triplet excited state energies. Basis sets were as follows: silver CEP-121G,41–43 phosphorus, fluorine, nitrogen 6-31G**, carbon 6-31G*, and hydrogen 6-31G. Population analysis was carried out by the AOMIX software.44 The pictures of the orbitals have been depicted with Molekel software.45
Acknowledgements
We thank Prof. S. Satokawa and Dr N. Shimoda of Seikei University for the measurement of Powder X-ray diffraction. This work was financially supported by the Grants from the Faculty of Science and Technology, Seikei University.
Notes and references
- H. M. Lee and S. P. Nolan, Org. Lett., 2000, 2, 2053–2055 CrossRef CAS PubMed.
- H. Kaur, F. K. Zinn, E. D. Stevens and S. P. Nolan, Organometallics, 2004, 23, 1157–1160 CrossRef CAS.
- M. S. Viciu, R. F. Germaneau and S. P. Nolan, Org. Lett., 2002, 4, 4053–4056 CrossRef CAS PubMed.
- R. Visbal and M. C. Gimeno, Chem. Soc. Rev., 2014, 43, 3551 RSC.
- L. Mercs and M. Albrecht, Chem. Soc. Rev., 2010, 39, 1903–1912 RSC.
- P. J. Barnard, L. E. Wedlock, M. V. Baker, S. J. Berners-Price, D. A. Joyce, B. W. Skelton and J. H. Steer, Angew. Chem., Int. Ed., 2006, 45, 5966–5970 CrossRef CAS PubMed.
- V. J. Catalano and M. A. Malwitz, Inorg. Chem., 2003, 42, 5483–5485 CrossRef CAS PubMed.
- V. J. Catalano and A. O. Etogo, Inorg. Chem., 2007, 46, 5608–5615 CrossRef CAS PubMed.
- C.-H. Yang, J. Beltran, V. Lemaur, J. Cornil, D. Hartmann, W. Sarfert, R. Fröhlich, C. Bizzarri and L. De Cola, Inorg. Chem., 2010, 49, 9891–9901 CrossRef CAS PubMed.
- Z. M. Hudson, C. Sun, M. G. Helander, Y.-L. Chang, Z.-H. Lu and S. Wang, J. Am. Chem. Soc., 2012, 134, 13930–13933 CrossRef CAS PubMed.
- X.-W. Li, H.-Y. Li, G.-F. Wang, F. Chen, Y.-Z. Li, X.-T. Chen, Y.-X. Zheng and Z.-L. Xue, Organometallics, 2012, 31, 3829–3835 CrossRef CAS.
- V. A. Krylova, P. I. Djurovich, M. T. Whited and M. E. Thompson, Chem. Commun., 2010, 46, 6696–6698 RSC.
- C.-S. Lee, S. Sabiah, J.-C. Wang, W.-S. Hwang and I. J. B. Lin, Organometallics, 2010, 29, 286–289 CrossRef CAS.
- Y. Zhang, J. A. Garg, C. Michelin, T. Fox, O. Blacque and K. Venkatesan, Inorg. Chem., 2011, 50, 1220–1228 CrossRef CAS PubMed.
- K. M. Lee, H. M. J. Wang and I. J. B. Lin, J. Chem. Soc., Dalton Trans., 2002, 2852–2856 RSC.
- V. J. Catalano and A. L. Moore, Inorg. Chem., 2005, 44, 6558–6566 CrossRef CAS PubMed.
- T. Sajoto, P. I. Djurovich, A. Tamayo, M. Yousufuddin, R. Bau, M. E. Thompson, R. J. Holmes and S. R. Forrest, Inorg. Chem., 2005, 44, 7992–8003 CrossRef CAS PubMed.
-
M. Thompson, P. Djurovich and V. Krylova, U.S. Patent Appl, 12/948396, 2013 Search PubMed.
-
(a) K. Matsumoto, T. Shindo, N. Mukasa, T. Tsukuda and T. Tsubomura, Inorg. Chem., 2010, 49, 805–814 CrossRef CAS PubMed;
(b) M. Osawa and M. Hoshino, Chem. Commun., 2008, 6384–6386 RSC;
(c) S. Igawa, M. Hashimoto, I. Kawata, M. Hoshino and M. Osawa, Inorg. Chem., 2012, 51, 5805–5813 CrossRef CAS PubMed.
- H. M. J. Wang and I. J. B. Lin, Organometallics, 1998, 17, 972–975 CrossRef CAS.
-
(a) C.-F. Chang, Y.-M. Cheng, Y. Chi, Y.-C. Chiu, C.-C. Lin, G.-H. Lee, P.-T. Chou, C.-C. Chen, C.-H. Chang and C.-C. Wu, Angew. Chem., Int. Ed., 2008, 47, 4542–4545 CrossRef CAS PubMed;
(b) T. Hofbeck, U. Monkowius and H. Yersin, J. Am. Chem. Soc., 2015, 137, 399–404 CrossRef CAS PubMed.
- B. R. Crenshaw and C. Weder, Chem. Mater., 2003, 15, 4717–4724 CrossRef CAS.
- S. Mizukami, H. Houjou, K. Sugaya, E. Koyama, H. Tokuhisa, T. Sasaki and M. Kanesato, Chem. Mater., 2005, 17, 50–56 CrossRef CAS.
-
(a) T. Tsukuda, M. Kawase, A. Dairiki, K. Matsumoto and T. Tsubomura, Chem. Commun., 2010, 46, 1905–1907 RSC;
(b) T. Seki, T. Ozaki, T. Okura, K. Asakura, A. Sakon, H. Uekusa and H. Ito, Chem. Sci., 2015, 6, 2187–2195 RSC;
(c) T. Abe, T. Itakura, N. Ikeda and K. Shinozaki, Dalton Trans., 2009, 711–715 RSC;
(d) A. L. Balch, Angew. Chem., Int. Ed., 2009, 48, 2641–2644 CrossRef CAS PubMed.
- T. Wen, D.-X. Zhang, H.-X. Zhang, H.-B. Zhang, J. Zhang and D.-S. Li, Chem. Commun., 2014, 50, 8754–8756 RSC.
- D. V. Partyka and N. Deligonul, Inorg. Chem., 2009, 48, 9463 CrossRef CAS PubMed.
- A. Cingolani, J. V. Hanna, M. Pellei, C. Pettinari, C. Santini, B. W. Skelton and A. H. White, Inorg. Chem., 2003, 42, 4938–4948 CrossRef CAS PubMed.
- M. Osawa, Chem. Commun., 2014, 50, 1801–1803 RSC.
- B. R. Crenshaw and C. Weder, Chem. Mater., 2003, 15, 4717–4724 CrossRef CAS.
- X. Shan, H. Zhang, L. Chen, M. Wu, F. Jiang and M. Hong, Cryst. Growth Des., 2013, 13, 1377–1381 CAS.
- S. Ito, T. Itoh and M. Nakamura, Angew. Chem., Int. Ed., 2011, 50, 454–457 CrossRef CAS PubMed.
- G. R. Fulmer, A. J. M. Miller, N. H. Sherden, H. E. Gottlieb, A. Nudelman, B. M. Stoltz, J. E. Bercaw and K. I. Goldberg, Organometallics, 2010, 29, 2176–2179 CrossRef CAS.
- M. Nishikawa, Y. Wakita, T. Nishi, T. Miura and T. Tsubomura, Dalton Trans., 2015, 44, 9170 RSC.
- K. Suzuki, A. Kobayashi, S. Kaneko, K. Takehira, T. Yoshihara, H. Ishida, Y. Shiina, S. Oishi and S. Tobita, Phys. Chem. Chem. Phys., 2009, 11, 9850 RSC.
-
Rigaku, CrystalClear, Rigaku Corporation, Tokyo, Japan, 2000 Search PubMed.
- A. Altomare, G. Cascarano, C. Giacovazzo, A. Guagliardi, M. C. Burla, G. Polidori and M. Camalli, J. Appl. Crystallogr., 1994, 27, 435–436 Search PubMed.
- G. M. Sheldrick, Acta Crystallogr., Sect. A: Fundam. Crystallogr., 2007, 64, 112–122 CrossRef PubMed.
- L. J. Farrugia, J. Appl. Crystallogr., 1999, 32, 837–838 CrossRef CAS.
-
M. J. Frisch, G. W. Trucks, H. B. Schlegel, G. E. Scuseria, M. A. Robb, J. R. Cheeseman, J. A. Montgomery, Jr., T. Vreven, K. N. Kudin, J. C. Burant, J. M. Millam, S. S. Iyengar, J. Tomasi, V. Barone, B. Mennucci, M. Cossi, G. Scalmani, N. Rega, G. A. Petersson, H. Nakatsuji, M. Hada, M. Ehara, K. Toyota, R. Fukuda, J. Hasegawa, M. Ishida, T. Nakajima, Y. Honda, O. Kitao, H. Nakai, M. Klene, X. Li, J. E. Knox, H. P. Hratchian, J. B. Cross, V. Bakken, C. Adamo, J. Jaramillo, R. Gomperts, R. E. Stratmann, O. Yazyev, A. J. Austin, R. Cammi, C. Pomelli, J. W. Ochterski, P. Y. Ayala, K. Morokuma, G. A. Voth, P. Salvador, J. J. Dannenberg, V. G. Zakrzewski, S. Dapprich, A. D. Daniels, M. C. Strain, O. Farkas, D. K. Malick, A. D. Rabuck, K. Raghavachari, J. B. Foresman, J. V. Ortiz, Q. Cui, A. G. Baboul, S. Clifford, J. Cioslowski, B. B. Stefanov, G. Liu, A. Liashenko, P. Piskorz, I. Komaromi, R. L. Martin, D. J. Fox, T. Keith, M. A. Al-Laham, C. Y. Peng, A. Nanayakkara, M. Challacombe, P. M. W. Gill, B. Johnson, W. Chen, M. W. Wong, C. Gonzalez and J. A. Pople, Gaussian, Inc., Wallingford CT, 2004.
- J. P. Perdew, K. Burke and M. Ernzerhof, Phys. Rev. Lett., 1997, 78, 1396 CrossRef CAS.
- W. Stevens, H. Basch and J. Krauss, J. Chem. Phys., 1984, 81, 6026 CrossRef.
- W. J. Stevens, M. Krauss, H. Basch and P. G. Jasien, Can. J. Chem., 1992, 70, 612 CrossRef CAS.
- T. R. Cundari and W. J. Stevens, J. Chem. Phys., 1993, 98, 5555 CrossRef CAS.
-
S. I. Gorelsky, AOMix: Program for Molecular Orbital Analysis, University of Ottawa, 2011 Search PubMed.
-
U. Varetto, Molekel, Swiss National Supercomputing Centre, Lugano, Switzerland Search PubMed.
Footnote |
† Electronic supplementary information (ESI) available: Crystallographic data for X-ray structural analysis, NMR spectra, emission spectra, and details of the DFT calculations. CCDC 1472558 and 1472559. For ESI and crystallographic data in CIF or other electronic format see DOI: 10.1039/c6qi00254d |
|
This journal is © the Partner Organisations 2016 |
Click here to see how this site uses Cookies. View our privacy policy here.