Photocatalytic degradation of ibuprofen using TiO2 sensitized by Ru(II) polyaza complexes†
Received
23rd June 2016
, Accepted 5th September 2016
First published on 6th September 2016
Abstract
In this work, modification of TiO2 was carried out by incorporation of two novel Ru(II) polyaza complexes. The N1-(2-aminobenzyliden)-N2,N2-bis(2-(2-aminobenzyliden)aminoethyl)ethane-1,2-diaminoruthenium(II) and N1,N2-bis(2-aminobenziliden)ethane-1,2-diaminoruthenium(II) complexes were synthesized via metal–ligand direct reaction. The complexes were characterized by UV-Vis, FTIR and fluorescence spectroscopy, and the chemical composition was obtained from elemental analysis by the combustion method; additionally, the sensitized TiO2 catalysts were also characterized by XRD, SEM and diffuse reflectance techniques. The photocatalytic activity of the prepared catalysts was tested in a batch reactor under visible radiation for the degradation of ibuprofen in aqueous solution. The evolution of the drug degradation process was evaluated by high-performance liquid chromatography (HPLC), while the mineralization percentage was monitored by the determination of total organic carbon (TOC). The results indicated that the incorporation of these complexes improves the activation of TiO2 under visible light, increasing the degradation and mineralization percentage of ibuprofen up to 35% compared to the unmodified material, thereby making it suitable for application in heterogeneous photocatalysis of the said pharmaceutical in aqueous media using visible light as the energy source.
Introduction
Titanium dioxide (TiO2) has been the most studied catalyst due to its potential application in the field of wastewater treatment.1–3 However, the need for UV light to promote the electron transfer process arising from the wide TiO2 band gap (ca. 3.2 eV) is a serious limitation for the practical applications of TiO2 in heterogeneous photocatalysis. There has been increasing interest in discovering effective approaches for improving the photoresponse of TiO2 particles under irradiation with low energy photons.4–8 One of the most effective strategies is the sensitization of the semiconductor, by incorporation of coloured compounds, such as metal complexes that interact with the surface of a wide band gap semiconductor, e.g., TiO2, facilitating electron transfer between the incorporated molecules and the host semiconductor. In this case, visible light excites the sensitizer organic molecules adsorbed onto the semiconductor, thereby enabling electron injection into the semiconductor conduction band. Surface photosensitization by coordination metal complexes via photo-induced sensitizer-to-TiO2 charge transfer exhibits attractive features such as regenerative sensitization and the ability to mediate the degradation of non-visible absorbing substrates.9–11
The difficulty of establishing stable surface anchorage of the charge-transfer photosensitizers is an important drawback of the semiconductor photosensitization approach. In this context, ruthenium(II) complexes have been preferred for use as charge transfer sensitizers owing to their wider absorption band (400–800 nm wavelength range) compared to the metal-free organic sensitizers. Ruthenium(II) complexes are widely used as sensitizers12–17 in dye-sensitized solar cells and as catalysts or photocatalysts capable of performing water oxidation or oxidation of organic compounds; therefore, in this work, we proposed to apply two new polyaza Ru(II) complexes as TiO2 sensitizers because of their particular properties and their ability to bind efficiently to various types of substrates.18
The Ru(II)-complexes, denominated N1-(2-aminobenzyliden)-N2,N2-bis(2-(2-aminobenzyliden)aminoethyl)ethane-1,2-diaminoruthenium(II), and N1,N2-bis(2-aminobenziliden)ethane-1,2-diaminoruthenium(II), labelled as RuL2 and RuL3, respectively (Fig. 1), were synthesized and incorporated onto the TiO2 semiconductor in order to evaluate the effect of the sensitization on the photocatalytic activity of the modified TiO2 catalysts under visible light irradiation. The nonsteroidal anti-inflammatory drug ibuprofen (C13H18O2) in aqueous solution was used as a model compound to study the performance of the sensitized materials. Regarding ibuprofen degradation some studies reported the complete degradation of this drug using TiO2 or ZnO irradiation with UV lamps, which implies a high energy cost. In another case Feng et al.19 reported the degradation of ibuprofen with TiO2 under visible light, however they had to add H2O2 during the process in order to improve the degradation rate.20–28 In this context, it is important to remark that no studies have been reported on the degradation of ibuprofen under visible light using TiO2 sensitized with Ru(II) complexes in order to achieve an efficient process less dependent on UV energy.
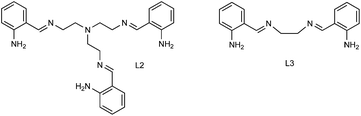 |
| Fig. 1 Structure of the polyaza ligands L2 and L3. | |
Experimental
Materials and reagents
RuCl3, ethylenediamine, 2-aminoethylamine, ethanol, methanol, ethyleneglycol, dimethylsulfoxide and ibuprofen ((RS)-2-(4-(2-methylpropyl)phenyl)propanoic acid) were supplied by Sigma-Aldrich. Titanium dioxide P25 was supplied by Degussa, in the form of 30% rutile and 70% anatase phases, with an average particle size of approximately 21 nm and a surface area of 50 m2 g−1. All solvents were used without further purification.
Characterization
Elemental analysis of the synthesized products was conducted using a Perkin-Elmer 2400 Series II CHNS/O elemental analyser. FTIR spectroscopy analysis was carried out using a Perkin-Elmer FT-IR Spectrometer Paragon 1000 PC. The diffuse reflectance analysis was performed using a Thermo-Scientific spectrophotometer model 300 PC coupled to an integrating sphere. The powder XRD patterns of the photocatalysts were recorded on a Siemens D-5000 diffractometer. The Scherrer formula was used to estimate the average crystallite size of the prepared materials from the data obtained from their X-ray diffraction patterns. The micrographs of the catalyst surfaces were obtained using a JEOL JSM-6510 Scanning Electron Microscope.
Complex synthesis
All synthesis reactions were carried out under constant stirring provided by a Fisher Sonic Dismembrator model 500. The two polyaza macroligands were synthesized by linking 2-aminobenzaldehyde with 2-tris-(2-aminoethyl)amine and ethylenediamine, respectively. The precursor 2-aminobenzaldehyde was obtained by reaction of 2-nitrobenzaldehyde with Fe0 in hydrochloric acid.29 The Ru(II) complexes were obtained at room temperature by direct reaction of the ligand and RuCl3, both in ethyleneglycol in a 1
:
1 mol ratio (M
:
L). Each reaction mixture was stirred for 30 min, filtered, washed with ethanol, and finally dried in a vacuum desiccator. The complexes were isolated as dark red solids and were labelled as RuL2 and RuL3.
Semiconductor modification
For the modification of the semiconductor, a suspension of 1 g of TiO2 in 1 L of water was prepared; separately a solution of each complex was prepared in 5 mL of dimethylsulfoxide. The incorporated complex amounts were 1 wt% and 1.5 wt% of both RuL2 and RuL3. The suspension and the complex solutions were sonicated for 30 min and then mixed and stirred for 3 h. Finally, the mixture was filtered and washed with 50 mL of acetone and 50 mL of water; the solids were recovered and dried prior to characterization by SEM, UV-Vis, FTIR and diffuse reflectance techniques.
Photocatalytic tests
Photocatalytic experiments were performed at room temperature. A quantity of 0.15 g of catalyst was added to a batch reactor containing 150 mL of 10 mg L−1 ibuprofen solution. A visible light metal halide Philips lamp (25 W) emitting polychromatic radiation in the 400–700 nm spectral range (with the maximum in the 540–620 nm wavelength range) was used to irradiate the solution. The light intensity received by the vessel was 50 mW cm2, as measured by using a HD 9221 radiometer with a spectral field from 450 to 950 nm. The photocatalytic reaction was performed under magnetic stirring at pH 6 and 8. Aliquots were taken every 30 min during the course of the degradation and filtered through a 0.45 μm nylon syringe filter, until the reaction time reached 300 min. The ibuprofen concentration decay was monitored by reversed-phase chromatography (Young-Lin model 9100) using an Ultra C18 Restek column (5 μm, 200 × 3 mm) and a photodiode array detector (Young-Lin 9160) at wavelength λ = 254 nm. The mobile phase was composed of methanol
:
0.1 M acetic acid in water (70
:
30) using a 1 mL min−1 flow rate. Mineralization was followed by TOC decay measurements performed using a Shimadzu VCSN analyser. Control adsorption experiments without irradiation and photolysis tests without catalyst were conducted in order to determine their contribution to the ibuprofen degradation. Additionally, reusing tests were carried out to establish the reusability cycles for the modified catalysts. These experiments were performed by recovering the catalyst after each cycle of use. The material was then filtered and washed with water and acetone and finally dried in a vacuum desiccator. All the experiments were performed in triplicate.
To determine the possibility of the release of the Ru(II) complexes in water, stability tests were carried out by suspending 0.15 g of modified catalyst in 150 mL of nanopure water. The suspension was vigorously stirred under visible light over 5 h, recording the UV-Vis spectra of the final solution and comparing with that of a Ru(II) complex suspension [Fig. SM1 and SM2†].
Results and discussion
The synthesized Ru(II)-complexes (RuL2 and RuL3) were crystalline powders, coloured, non-hygroscopic, and non-soluble in water. Examination of the results of the elemental analysis of the synthesized complexes (Table 1) shows that these were obtained successfully and according to the suggested chemical formula and structure. The most important data from the IR spectra (not shown) indicate that the two ruthenium(II) complexes were obtained, and also show that there is a shift to lower wavenumbers (higher bonding energy) for each signal, indicating an interaction between the N-heteroatoms and the Ru(II) in the coordination sphere.14,30,31
Table 1 Chemical properties of the synthesized Ru(II) complexes
Complex |
Empirical formula |
%C found (required) |
%H found (required) |
%N found (required) |
RuL2 |
C27H33N7Cl2Ru |
47.1 (47.5) |
4.3 (4.6) |
18.0 (18.3) |
RuL3 |
C16H18N4Cl2Ru |
38.8 (39.1) |
3.5 (3.7) |
16.7 (17.1) |
The X-ray diffraction patterns shown in Fig. 2 indicate that there is no significant change in the 2θ angle of the signals for the sensitized TiO2 catalysts compared to the unmodified TiO2 Degussa; however, there is an effect of peak widening mainly at 25.4°, 62.5° and 55° 2θ, indicating a difference in crystallite size for the modified materials. Therefore, the crystallite size of the catalyst was determined from these peaks using the Scherrer formula:
The results in
Table 2 indicate a decrease in the crystallite size as the Ru(
II)-complexes are incorporated on TiO
2. This same behaviour has been reported by Bahadur
et al.32 and Behnajady
et al.33 when metallic ions were incorporated by impregnation on the surface of the catalyst as a result of the interaction between the incorporated ion and TiO
2.
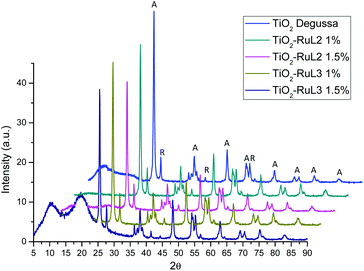 |
| Fig. 2 X-Ray diffraction patterns for each material. | |
Table 2 Average crystallite size and calculated Eg values for TiO2 modified catalysts
Catalyst |
E
g (eV) |
λ (nm) |
Average crystallite Size (nm) |
TiO2 |
3.2 |
387 |
25.03 |
TiO2-RuL2 1% |
3.0 |
413 |
22.31 |
TiO2-RuL2 1.5% |
2.9 |
427 |
21.15 |
TiO2-RuL3 1% |
3.1 |
400 |
22.69 |
TiO2-RuL3 1.5% |
3.1 |
400 |
21.94 |
The SEM micrographs for TiO2, TiO2-RuL2 and TiO2-RuL3 1.5% samples (Fig. 3) show a difference in the size of particles, ranging from 20–35 nm for pure TiO2 to 12–20 nm for the modified semiconductor. This decrease of particle size is also observed in the SEM images in agreement with the results obtained from the Scherrer equation; therefore, these results can indicate that the presence of the complexes on the surface of titania particles can enhance the surface area of the catalyst.34
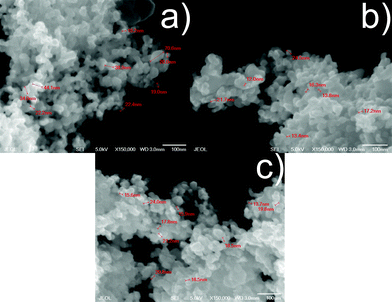 |
| Fig. 3 SEM images of (a) TiO2, (b) TiO2-RuL2 1.5% and (c) TiO2-RuL3 1.5%. | |
Fig. 4 shows the plots of (F(R)hν)1/2vs. hν of the modified photocatalysts and TiO2 Degussa. Band gap values were calculated for every material using the Kubelka–Munk function:
The absorption edge can be observed at approximately 3.2 eV, corresponding to a charge transfer process from the valence band to the conduction band of the semiconductor. This edge exhibits a displacement to lower energy values in the modified TiO
2, suggesting a narrowing of the band gap as a consequence of the Ru(
II)-complex incorporation.
35,36 The most noticeable displacement is found in the catalyst modified with RuL2 at 1.5 wt% that exhibits the lower
Eg value (2.9 eV) corresponding to the energy of the visible region (427 nm).
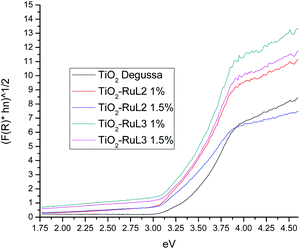 |
| Fig. 4 Diffuse reflectance spectra for TiO2 and sensitized TiO2 catalysts. | |
In Table 2, the Eg values and the crystallite size for each catalyst are reported. It is noticeable that the smaller crystallite size (21.15 nm) is also observed for TiO2-RuL2 1.5%. The lower Eg along with the small crystallite size, suggests that the catalyst TiO2-RuL2 1.5% can be an adequate material for the activation with visible light. These changes of the Eg value and the crystallite size are a direct consequence of the presence of the Ru(II) complexes on the surface of TiO2, which can enhance the photocatalytic activity under visible light.37–39
Photocatalytic experiments
The results shown in Fig. 5 indicate that a higher degradation percentage (∼80%) especially at pH 8 (Fig. 5b), was obtained using the sensitized materials with 1.5% of RuL2 and RuL3, surpassing the activity of unmodified TiO2. The isoelectric point of TiO2 (P25) is approximately 6.2,40 therefore at pH 8 the catalyst surface is mainly covered with negative charges Ti–O− which interact as an electron donor with O2 molecules generating more oxygen and hydroxyl radicals to oxidize the organic compounds.41–44 Also, at pH values higher than its pKa (5.2),45 the carboxylic group of ibuprofen reaches the ionized state which can form ion–dipole bonds with water and both its hydrophilicity and solubility are improved, hence, ibuprofen can easily reach the active sites of the catalyst, improving the degradation process.46–49
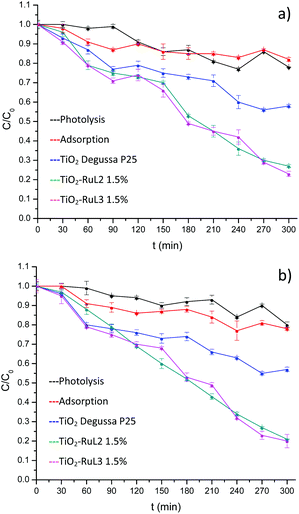 |
| Fig. 5 Photocatalytic activity of TiO2 and modified materials on the degradation of ibuprofen with visible light, at (a) pH 6 and (b) pH 8. | |
On the other hand, preliminary tests showed a poor performance in the case of the 1% complex modified catalysts, so that hereafter we only used modification with 1.5% complexes.
The photocatalytic degradation rate of organic compounds is commonly described in terms of the Langmuir–Hinshelwood model. It has been demonstrated in many reports50–53 that photocatalytic reactions can be adjusted to this pseudo-first order model in a low-concentration solution under direct irradiation of light. Most researchers continue using the L–H kinetic model for fitting the experimental results to a pseudo-first order kinetic expression for the condition kC ≪ 1 since it describes a simpler model for the representation of this process in engineering applications. Therefore, the photocatalytic degradation rate of organic compounds at low concentrations is commonly defined by the following equation:
where
C is the concentration of the reactant,
t is the irradiation time, and
k is the rate constant of the reaction.
The half-life (t1/2) for the decrease of half of the concentration of ibuprofen was calculated by:
The degradation rate constant (
k) was obtained using the slope from the linear fit of ln(
C0/
C)
vs. t. The kinetic parameters for the degradation process are shown in
Table 3. It is clear that the catalyst modified with 1.5% of the RuL2 complex exhibits better photocatalytic activity for the degradation of ibuprofen under visible light irradiation, achieving a higher rate constant (5.5 × 10
−3 min
−1) and a lower half-life time (126 min) of the oxidation process. No significant difference in the degradation percentage was observed for both modified catalysts. However, a significant difference is observed for the mineralization process.
Table 3 Kinetic parameters for the photocatalytic degradation of ibuprofen
|
pH 6 |
pH 8 |
Material |
k
app (min−1) |
t
1/2 (min) |
k
app (min−1) |
t
1/2 (min) |
TiO2-RuL2 1.5% |
3.9 × 10−3 |
177 |
5.5 × 10−3 |
126 |
TiO2-RuL3 1.5% |
3.7 × 10−3 |
187 |
3.8 × 10−3 |
182 |
Fig. 6 shows the TOC decay values for photocatalytic experiments, at the times of 0, 90, 180 and 300 min; it can be observed that a higher mineralization percentage (64%) is achieved with the RuL2 1.5% modified catalyst, in contrast with the mineralization percentage reached with RuL3 1.5% (45%). This difference can be attributed to the more flexible and complex structure of RuL2 that enables it to anchor to the TiO2 surface better and more easily than RuL3.
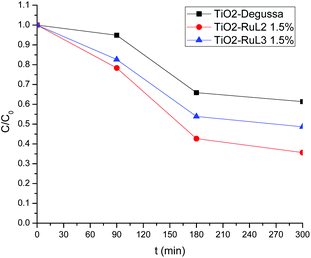 |
| Fig. 6 TOC decay on the degradation of ibuprofen with visible light at pH 8. | |
This higher mineralization percentage could indicate that, the route for the ibuprofen decomposition yields organic molecules with lower molecular weight and better solubility than ibuprofen, such as decarboxylated and hydroxylated metabolites, as well as organic acids.54
The selected TiO2-RuL2 material was recovered to study its ability to be reused in the degradation of ibuprofen through four degradation cycles. While the degradation capacity does not change greatly during the first and second cycle, after the third cycle this capacity decreases by 20%, probably because of saturation of the surface by the formed by-products during the photocatalytic process. On the other hand, the stability tests demonstrated that the prepared catalysts are stable since the UV-Vis absorption bands of the Ru(II)-complex were not detected in the spectra of the final solution of the mentioned tests. This result suggests that the Ru(II) complexes can be anchored to the TiO2 surface, allowing electronic transfer from the visible-light excited state of the complex to the conduction band of TiO2 resulting in effective electron injection and therefore, in the activation of the catalyst with low energy radiation.55
Conclusions
The enhancement of the photocatalytic properties of the sensitized materials is attributed to the presence of the complexes on the semiconductor surface that improves the absorption of visible light.
These results indicate that both Ru(II)-complexes (RuL2 and RuL3) can act as sensitizers; more importantly, in the case of RuL2, the complex incorporated at 1.5%, as was revealed by the higher ibuprofen mineralization percentage.
These sensitizers promote the activation of TiO2 under visible light irradiation by injecting electronic charge into the conduction band of the semiconductor and avoiding the electron–hole recombination.
Acknowledgements
The authors would like to acknowledge the Faculty of Chemistry of the Autonomous University of Nuevo León, Monterrey, México, and J. F. Góngora is grateful to the CONACYT for the scholarship granted for this project, and also to the CONACYT Project No. CB-181057.
References
- N. De la Cruz, R. F. Dantas, J. Giménez and S. Esplugas, Photolysis and TiO2 photocatalysis of the pharmaceutical propranolol: Solar and artificial light, Appl. Catal., B, 2013, 130–131, 249–256 CrossRef CAS.
- A. L. Giraldo,
et al. Degradation of the antibiotic oxolinic acid by photocatalysis with TiO2 in suspension, Water Res., 2010, 44, 5158–5167 CrossRef CAS PubMed.
- F. Méndez-Arriaga, S. Esplugas and J. Giménez, Photocatalytic degradation of non-steroidal anti-inflammatory drugs with TiO2 and simulated solar irradiation, Water Res., 2008, 42, 585–594 CrossRef PubMed.
- D. Li, H. Haneda, S. Hishita and N. Ohashi, Visible-light-driven nitrogen-doped TiO2 photocatalysts: Effect of nitrogen precursors on their photocatalysis for decomposition of gas-phase organic pollutants, Mater. Sci. Eng., B, 2005, 117, 67–75 CrossRef.
- E. S. Elmolla and M. Chaudhuri, Photocatalytic degradation of amoxicillin, ampicillin and cloxacillin antibiotics in aqueous solution using UV/TiO2 and UV/H2O2/TiO2 photocatalysis, Desalination, 2010, 252, 46–52 CrossRef CAS.
- S. Nakade,
et al. Influence of TiO2 Nanoparticle Size on Electron Diffusion and Recombination in Dye-Sensitized TiO2 Solar Cells, J. Phys. Chem. B, 2003, 107, 8607–8611 CrossRef CAS.
- A. Fujishima, X. Zhang and D. A. Tryk, TiO2 photocatalysis and related surface phenomena, Surf. Sci. Rep., 2008, 63, 515–582 CrossRef CAS.
- C. Belver, R. Bellod, A. Fuerte and M. Fernández-García, Nitrogen-containing TiO2 photocatalysts. Part 1. Synthesis and solid characterization, Appl. Catal., B, 2006, 65, 301–308 CrossRef CAS.
- H. Park, Y. Park, W. Kim and W. Choi, Surface modification of TiO2 photocatalyst for environmental applications, J. Photochem. Photobiol., C, 2013, 15, 1–20 CrossRef CAS.
- M. A. Henderson, A surface science perspective on TiO2 photocatalysis, Surf. Sci. Rep., 2011, 66, 185–297 CrossRef CAS.
- C. Di Valentin,
et al. N-doped TiO2: Theory and experiment, Chem. Phys., 2007, 339, 44–56 CrossRef CAS.
- P. Kar,
et al. Sensitization of nanocrystalline TiO2 anchored with pendant catechol functionality using a new tetracyanato ruthenium(II) polypyridyl complex, Inorg. Chem., 2010, 49, 4167–4174 CrossRef CAS PubMed.
- J. Warnan,
et al. Ruthenium sensitizer functionalized by acetylacetone anchoring groups for dye-sensitized solar cells, J. Phys. Chem. C, 2013, 117, 8652–8660 CAS.
- N. Onozawa-Komatsuzaki,
et al. Near-IR sensitization of nanocrystalline TiO2 with a new ruthenium complex having a 2,6-bis(4-carboxyquinolin-2-yl)pyridine ligand, Inorg. Chem. Commun., 2009, 12, 1212–1215 CrossRef CAS.
- T. Funaki,
et al. Ruthenium (II) complexes with expanded ligand having phenylene-ethynylene moiety as sensitizers for dye-sensitized solar cells, Sol. Energy Mater. Sol. Cells, 2009, 93, 729–732 CrossRef CAS.
- S. Fan, C. Kim, B. Fang and K. Liao, Improved efficiency of over 10% in dye-sensitized solar cells with a ruthenium complex and an organic dye heterogeneously positioning on a single TiO2 electrode, J. Phys. Chem. C, 2011, 7747–7754 CAS.
- W. Kaim, Concepts for metal complex chromophores absorbing in the near infrared, Coord. Chem. Rev., 2011, 255, 2503–2513 CrossRef CAS.
- P. Giokas and S. Miller, Spectroscopy and Dynamics of Phosphonate-Derivatized Ruthenium Complexes on TiO2, J. Phys. Chem. C, 2013, 117, 812–824 CAS.
- L. Feng, E. D. Van Hullebusch, M. A. Rodrigo, G. Esposito and M. A. Oturan, Removal of residual anti-inflammatory and analgesic pharmaceuticals from aqueous systems by electrochemical advanced oxidation processes. A review, Chem. Eng. J., 2013, 228, 944–964 CrossRef CAS.
- J. Kim, H. Jang and J. Kim, Occurrence of pharmaceutical and personal care products (PPCPs) in surface water from Mankyung River, South Korea, J. Health Sci., 2009, 55, 249–258 CrossRef CAS.
- R. Reif, S. Suárez, F. Omil and J. M. Lema, Fate of pharmaceuticals and cosmetic ingredients during the operation of a MBR treating sewage, Desalination, 2008, 221, 511–517 CrossRef CAS.
- S. Ortiz de García, G. Pinto Pinto, P. García Encina and R. Irusta Mata, Consumption and occurrence of pharmaceutical and personal care products in the aquatic environment in Spain, Sci. Total Environ., 2013, 444, 451–465 CrossRef PubMed.
- W.-J. Sim,
et al. Occurrence and distribution of pharmaceuticals in wastewater from households, livestock farms, hospitals and pharmaceutical manufactures, Chemosphere, 2011, 82, 179–186 CrossRef CAS PubMed.
- J. Rivera-Utrilla, M. Sánchez-Polo, M. Á. Ferro-García, G. Prados-Joya and R. Ocampo-Pérez, Pharmaceuticals as emerging contaminants and their removal from water. A review, Chemosphere, 2013, 93, 1268–1287 CrossRef CAS PubMed.
- J. B. Ellis, Pharmaceutical and personal care products (PPCPs) in urban receiving waters, Environ. Pollut., 2006, 144, 184–189 CrossRef CAS PubMed.
- O. A. Jones, J. N. Lester and N. Voulvoulis, Pharmaceuticals: A threat to drinking water?, Trends Biotechnol., 2005, 23, 163–167 CrossRef CAS PubMed.
- T. E. Félix-Cañedo, J. C. Durán-Álvarez and B. Jiménez-Cisneros, The occurrence and distribution of a group of organic micropollutants in Mexico City's water sources, Sci. Total Environ., 2013, 454–455, 109–118 CrossRef PubMed.
- J.-L. Liu and M.-H. Wong, Pharmaceuticals and personal care products (PPCPs): a review on environmental contamination in China, Environ. Int., 2013, 59, 208–224 CrossRef CAS PubMed.
- J. F. Góngora-Gómez,
et al. Sensitization of TiO2 with novel Cu(II) and Ni(II) polyaza complexes: Evaluation of its photocatalytic activity, Ceram. Int., 2014, 40, 14207–14214 CrossRef.
- X.-H. Wei,
et al. Two sensitizers of Tb(III) constructed from alkaline earth metals and semi-rigid tripodal ligand, Inorg. Chim. Acta, 2014, 416, 207–214 CrossRef CAS.
- T. Suresh,
et al. Novel ruthenium sensitizer with multiple butadiene equivalent thienyls as conjugation on ancillary ligand for dye-sensitized solar cells, Org. Electron., 2013, 14, 2243–2248 CrossRef CAS.
- N. Bahadur, K. Jain, R. Pasricha, Govind and S. Chand, Selective gas sensing response from different loading of Ag in sol–gel mesoporous titania powders, Sens. Actuators, B, 2011, 159, 112–120 CrossRef CAS.
- M. A. Behnajady and H. Eskandarloo, Silver and copper co-impregnated onto TiO2-P25 nanoparticles and its photocatalytic activity, Chem. Eng. J., 2013, 228, 1207–1213 CrossRef CAS.
- A. Kruth, A. Quade, V. Brüser and K. Weltmann, Plasma-Enhanced Synthesis of Poly (allylamine)-Encapsulated Ruthenium Dye-Sensitized Titania Photocatalysts, J. Phys. Chem. C, 2013, 117, 3804–3811 CAS.
- C. Martínez, L. M. Canle, M. I. Fernández, J. A. Santaballa and J. Faria, Aqueous degradation of diclofenac by heterogeneous photocatalysis using nanostructured materials, Appl. Catal., B, 2011, 107, 110–118 CrossRef.
- T. Morikawa, R. Asahi, T. Ohwaki, K. Aoki and Y. Taga, Band-gap narrowing of titanium dioxide by nitrogen doping, Jpn. J. Appl. Phys., Part 2, 2001, 40, L561–L563 CAS.
- A. V. Emeline, V. N. Kuznetsov, V. K. Rybchuk and N. Serpone, Visible-Light-Active Titania Photocatalysts: The Case of N-Doped TiO2—Properties and Some Fundamental Issues, Int. J. Photoenergy, 2008, 2008, 1–19 CrossRef.
- D. G. Brown, P. A. Schauer, J. Borau-Garcia, B. R. Fancy and C. P. Berlinguette, Stabilization of ruthenium sensitizers to TiO2 surfaces through cooperative anchoring groups, J. Am. Chem. Soc., 2013, 135, 1692–1695 CrossRef CAS PubMed.
- S. Banerjee,
et al. New Insights into the Mechanism of Visible Light Photocatalysis, J. Phys. Chem. Lett., 2014, 5, 2543–2554 CrossRef CAS PubMed.
- K. Suttiponparnit,
et al. Role of Surface Area, Primary Particle Size, and Crystal Phase on Titanium Dioxide Nanoparticle Dispersion Properties, Nanoscale Res. Lett., 2011, 6, 1–8 Search PubMed.
- Y. Fu and W. Cao, The effect of potential on the electron-trapping process of surface states in nanocrystalline TiO2 film electrode, J. Appl. Phys., 2006, 100, 084324 CrossRef.
- M. Buchalska,
et al. On Oxygen Activation at Rutile- and Anatase-TiO2, ACS Catal., 2015, 5, 7424–7431 CrossRef CAS.
- A. Imanishi, T. Okamura, N. Ohashi, R. Nakamura and Y. Nakato, Mechanism of water photooxidation reaction at atomically flat TiO 2 (rutile) (110) and (100) surfaces: Dependence on solution pH, J. Am. Chem. Soc., 2007, 129, 11569–11578 CrossRef CAS PubMed.
- A. Tsujiko,
et al. Observation of cathodic photocurrents at nanocrystalline TiO2 film electrodes, caused by enhanced oxygen reduction in alkaline solutions, J. Phys. Chem. B, 2002, 106, 5878–5885 CrossRef CAS.
- T. Loftsson and M. E. Brewster, Pharmaceutical applications of cyclodextrins: Drug solubilisation and stabilization, J. Pharm. Sci., 1996, 85, 1017–1025 CrossRef CAS PubMed.
- I. Vergili, Application of nanofiltration for the removal of carbamazepine, diclofenac and ibuprofen from drinking water sources, J. Environ. Manage., 2013, 127, 177–187 CrossRef CAS PubMed.
- F. Méndez-Arriaga, M. I. Maldonado, J. Gimenez, S. Esplugas and S. Malato, Abatement of ibuprofen by solar photocatalysis process: Enhancement and scale up, Catal. Today, 2009, 144, 112–116 CrossRef.
- R. Baccar, M. Sarr, J. Bouzid, M. Feki and P. Blánquez, Removal of pharmaceutical compounds by activated carbon prepared from agricultural by-product, Chem. Eng. J., 2012, 211–212, 310–317 CrossRef CAS.
- E. Illés,
et al. Hydroxyl radical induced degradation of ibuprofen, Sci. Total Environ., 2013, 447, 286–292 CrossRef PubMed.
- D. Dimitrakopoulou,
et al. Degradation, mineralization and antibiotic inactivation of amoxicillin by UV-A/TiO2 photocatalysis, J. Environ. Manage., 2012, 98, 168–174 CrossRef CAS PubMed.
- Z. Khuzwayo and E. M. N. Chirwa, Modelling and simulation of photocatalytic oxidation mechanism of chlorohalogenated substituted phenols in batch systems: Langmuir-Hinshelwood approach, J. Hazard. Mater., 2015, 300, 459–466 CrossRef CAS PubMed.
- D. WANG, Experimental Conditions for Valid Langmuir-Hinshelwood Kinetics, Chin. J. Catal., 2010, 31, 972–978 CrossRef CAS.
- M. L. Maya-Treviño, M. Villanueva-Rodríguez, J. L. Guzmán-Mar, L. Hinojosa-Reyes and A. Hernández-Ramírez, Comparison of the solar photocatalytic activity of ZnO-Fe2O3 and ZnO-Fe(0) on 2,4-D degradation in a CPC reactor, Photochem. Photobiol. Sci., 2015, 14, 543–549 Search PubMed.
- F. Méndez-Arriaga, S. Esplugas and J. Giménez, Degradation of the emerging contaminant ibuprofen in water by photo-Fenton, Water Res., 2010, 44, 589–595 CrossRef PubMed.
- S. Malato, P. Fernández-Ibáñez, M. I. Maldonado, J. Blanco and W. Gernjak, Decontamination and disinfection of water by solar photocatalysis: Recent overview and trends, Catal. Today, 2009, 147, 1–59 CrossRef CAS.
Footnote |
† Electronic supplementary information (ESI) available. See DOI: 10.1039/c6pp00222f |
|
This journal is © The Royal Society of Chemistry and Owner Societies 2017 |
Click here to see how this site uses Cookies. View our privacy policy here.