DOI:
10.1039/C6OB01817C
(Paper)
Org. Biomol. Chem., 2016,
14, 10011-10017
Strain-promoted azide–alkyne cycloaddition for protein–protein coupling in the formation of a bis-hemoglobin as a copper-free oxygen carrier†
Received
19th August 2016
, Accepted 27th September 2016
First published on 27th September 2016
Abstract
Conventional chemical approaches to protein–protein coupling present challenges due to the intrinsic competition between the desired interactions of reagents with groups of the protein as well as reactions with water. Biorthogonal Cu(I)-catalyzed azide–alkyne cycloaddition (CuAAC)-processes provide a basis to direct reactivity without functional group interference. However, the requirement for Cu(I) in CuAAC leads to complications that result from the metal ion's interactions with the protein. In principle, a similar but metal-free alternative approach to coupling could employ the reaction of an alkyne that is strained in combination with an azide (strain-promoted azide–alkyne cycloaddition, SPAAC). The method is exemplified by the combination of a cyclooctyne derivative of hemoglobin with an azide-modified hemoglobin. The bis-hemoglobin tetramer that is produced has properties consistent with those sought for use as a hemoglobin-based oxygen carrier (HBOC).
Introduction
Chemical cross-linking of individual proteins is a highly developed area with many applications and examples.1–4 Extending the approach that creates linkages within proteins to protein–protein coupling can produce novel entities with designed properties.2,5,6 Constructs of two hemoglobin (Hb) proteins coupled together, known as Hb bis-tetramers, have been found useful as circulating oxygen carriers that avoid extravasation from the vasculature.6 Their propensity to evade scavenging of nitric oxide from endothelial nitric oxide synthase7 supports their potential utility and value as protein-based synthetic targets. However, because proteins have many reactive functional groups, directing a specific coupling reaction to unique sites is a chemical challenge. Furthermore, developing reactions between proteins confronts a significant entropic challenge. The concept and implementation of biorthogonal processes led to the successful application of copper-catalyzed azide–alkyne cycloaddition (CuAAC)8 in the formation of useful coupled proteins.9–11 For example, we adapted CuAAC to coupling stabilized Hb tetramers using the solubility-directed combination of cross-linked Hb azides and bis-alkynes in the presence of Cu(I).10,12,13 The process is also applicable to coupling different proteins and can serve as the basis for a more general approach to coupling proteins.9 However, the requirement for Cu(I) can lead to protein denaturation and the ongoing association of protein-bound Cu(I).14,15 Subsequent release of copper in circulation is then concerning due to its capacity to interfere with cellular processes.16 However, the concentrations of copper will be small as we purified the products with this issue in mind.
Limitations that result from complications associated with CuAAC17–19 motivated us to develop an alternative biorthogonal coupling that does not require an added metal ion. Bertozzi and co-workers have shown that cycloalkynes are activated for such a process, presumably by strain that is induced by distortion of their triple bond.20,21 We now report that using strain-promoted azide–alkyne cycloaddition (SPAAC) as a metal-free alternative,22–26 we can efficiently produce cross-linked Hb bis-tetramers. The preparation of the necessary modified proteins for the cycloaddition process is convenient and the controlled cycloadditions give readily isolable-coupled proteins as products.
Experimental section
Materials
Human hemoglobin A (HbA) was obtained from Oxygenix Ltd. N-[(1R,8S,9S)-Bicyclo[6.1.0]non-4-yn-9-ylmethyloxycarbonyl]-1,8-diamino-3,6-dioxaoctane (amine-cyclooctyne), 3,6,9,12,15-pentaoxaheptadecane-1,17-diyl bis-azide and amino-PEG4-alkyne (amine-alkyne) were obtained from commercial sources. Trimesoyl tris(3,5-dibromosalicylate) (TTDS) was synthesized according to Kluger et al.27 4-Azidomethyl-benzylamine (amine-azide) and the reference bis-tetramer were prepared as described by Yang et al.12 4,4′-Diazidediphenylsulfone (bis-azide) was synthesized according to Li et al.17
Native gel electrophoresis
Two-dimensional Tris-HCl polyacrylamide gels contained 12% separating gel (pH 8.8) and 4% stacking gel (pH 8.8). Sample buffer was adjusted to pH 6.8 and running buffer was adjusted to pH 8.3. The finished gels were stained with Coomassie Brilliant Blue. Polyacrylamide gel electrophoresis (PAGE) followed standard operations.28
Hb bis-tetramers by SPAAC
Preparation of Hb–cyclooctyne.
A solution of Hb (0.5 mM in 1.5 mL of 50 mM sodium borate buffer, pH 9.0) was oxygenated by stirring under a stream of oxygen with photoirradiation for 2 h at 4 °C. The sample was then deoxygenated by stirring under a stream of nitrogen for 2 h at 37 °C. TTDS was added (2.0 eq. of 0.2 M solution in DMSO) and then stirred for 12 min. Amine-cyclooctyne was added (40 eq. of 1.0 M solution in DMSO) and the sample flushed with carbon monoxide. After 1 h stirring at room temperature, the mixture was passed through a Sephadex G-25 column equilibrated with phosphate buffer (0.02 M, pH 7.4). The collected fractions were concentrated by centrifugation through a filter (30 kDa cut-off) and stored under carbon monoxide at 4 °C. The compositions of the products were analyzed by HPLC using a 330 Å C-4 Vydac reverse-phase column (4.6 mm × 250 mm) and a solvent gradient from 20 to 60% acetonitrile in water spiked with 0.1% trifluoroacetic acid. The eluent was monitored at 220 nm. Slight drifts in retention times were observed because solvents were mixed outside the system. The identities of the material in the resulting peaks were assigned using positive electrospray ionization high resolution mass spectrometry analysis (Agilent 6538 Q-TOF equipped with a de-salting column, AIMS Laboratory, Department of Chemistry, University of Toronto).
Preparation of Hb–azide.
The procedure is an optimization of the method reported by Yang et al.12 and is identical to the preparation of Hb–cyclooctyne with the following exceptions: amine-azide was added instead of the amine-cyclooctyne described above. The mixture was passed through a column of Sephadex G-25 that had been equilibrated with MOPS buffer (0.1 M, pH 8.0). The products were analyzed by reverse-phase HPLC and their mass spectra.
SPAAC of Hb–cyclooctyne with Hb–azide.
Hb–cyclooctyne (1.0 eq., 100 μL of a 0.32 mM stock solution in 0.02 M phosphate buffer, pH 7.4) was combined with Hb–azide (1 eq., 133 μL of a 0.24 mM stock solution in 0.02 M phosphate buffer, pH 7.4). The total volume of the reaction mixture was adjusted to 100 μL by concentration through a membrane (30 kDa cut-off). This solution was incubated for 12 days at 4 °C under an atmosphere of carbon monoxide. The products were analyzed by HPLC using a Superdex G-200 HR size-exclusion column (10 mm × 300 mm) and tris-HCl (37.5 mM, pH 7.4) elution buffer containing magnesium chloride (0.5 M). The eluent was monitored at 280 nm.
SPAAC of Hb–cyclooctyne with bis-azide.
To Hb–cyclooctyne (1 eq., 100 μL of a 0.32 mM stock solution in 0.02 M phosphate buffer, pH 7.4) was added 0.45 eq. of bis-azide (4.8 μL of a 3 mM stock solution in DMSO). The mixture was incubated for 15 days at 4 °C under an atmosphere of carbon monoxide. The products were analyzed by size-exclusion HPLC and mass spectrometry as described above. The bis-tetramer was separated from the reactants by passing the mixture through a Sephadex G-100 column equilibrated with Tris-HCl (37.5 mM, pH 7.4) containing magnesium chloride (0.5 M). The first fraction, containing the purified bis-tetramer, was concentrated through a membrane (30 kDa cut-off) and stored under an atmosphere of carbon monoxide at 4 °C. The composition of the purified bis-tetramer was evaluated by size-exclusion HPLC as previously described.
Oxygen binding.
The oxygen pressure at half-saturation (P50) and the Hill's coefficient of cooperativity at half-saturation (n50) of the purified bis-tetramer were determined using a Hemox Analyzer™ with the sample maintained at 27 °C. These conditions were optimized for laboratory measurements and not as a model for circulatory studies. Hb samples (5 mL, 0.013 M), prepared in phosphate buffer (0.01 M, pH 7.4), were oxygenated prior to analysis by stirring under a stream of oxygen with photoirradiation for 1.5 h at 4 °C. The sample was then contained in a cell connected to the Hemox Analyzer™ for acquisition of the oxygen desaturation curve. The conversion to the deoxy state was achieved by flushing the cell with nitrogen. The data were fitted to the Adair equation using computation of a best fit by the method of non-linear least squares.
Results and discussion
Human Hb was cross-linked with trimesoyl tris(3,5-dibromosalicylate) (Scheme 1), a trifunctional reagent that reacts with the ε-amino groups of each β-lys-82, leaving the third ester available for further reaction.27,29 The polyanionic electrophile reacts site-specifically with residues residing within Hb's cationic funnel that normally associate with 2,3-diphosphoglycerate. Addition of an amine-functionalized hydrocarbon derivative of cyclooctyne30 to the cross-linked protein ester produces the desired conjugate (also in Scheme 1). Addition of an amino azide to the cross-linked protein ester produces an azido conjugate (Scheme 2). These derivatives were characterized by reverse-phase HPLC of the product solutions. Fig. 1 shows the chromatogram for the reaction products from the Hb–cyclooctyne conjugation. Fig. 2 shows the chromatogram for formation of the Hb–azide conjugate. Both samples contained small amounts of non-conjugated products that result from a side reaction that is typically due to competitive hydrolysis of the initial ester.
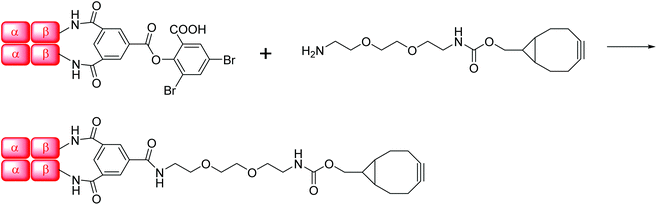 |
| Scheme 1 Preparation of Hb–cyclooctyne. | |
 |
| Scheme 2 Preparation of Hb–azide. | |
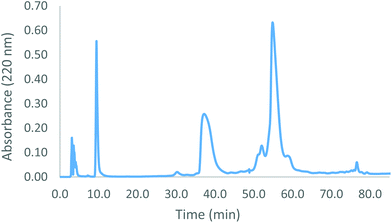 |
| Fig. 1 Reverse-phase HPLC trace of cross-linked Hb–cyclooctyne under dissociating conditions. Peaks are as follows: heme (10 min.); α-subunits (38 min.); cross-linked β-subunits (hydrolysis product, 52 min.); cross-linked β-subunits (cyclooctyne modified, 55 min.). | |
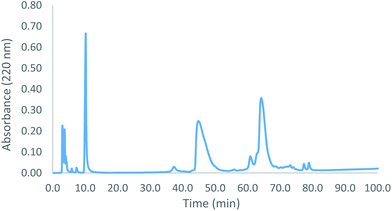 |
| Fig. 2 Reverse-phase HPLC trace of Hb–azide under dissociating conditions. Peaks are as follows: heme (10 min); α-subunits (45 min); cross-linked β-subunits (hydrolysis product, 62 min); cross-linked β-subunits (azide modified, 64 min). | |
The cycloaddition of Hb–azide and the Hb–cyclooctyne was initiated by combining solutions of each reactant (Scheme 3). Progress of the reaction was followed by HPLC until an optimal conversion was achieved. After 12 days the reactants were converted in 70% yield to the cycloaddition product, which is a cross-linked bis-tetramer connected by the triazole from the SPAAC process (Fig. 3). Gel electrophoretic analysis confirmed that the peak that elutes earlier than the modified Hb tetramers in the size-exclusion HPLC is the bis-tetrameric species (Fig. 6). Allowing the reaction to proceed for up to 18 more days did not increase the yield. Other modes of addition and combination of the reactants did not improve the final outcome. Notably, addition of amine-cyclooctyne/azide reagents to CO-Hbs to secure uniformity of the product conformation did not enhance the subsequent SPAAC reaction. The yield increases to 76% by keeping the mixed proteins in their deoxygenated states for four more days. We expect that access to the central channel of the protein is improved in the conformation favored by the deoxy heme.13 However, this is impractical for a long-term reaction because of the competing formation of non-functional methemoglobin from small amounts of residual oxygen. There was no added benefit neither from heating the solution at 70 °C for 30 min nor by stirring for 12 h at 40 °C.
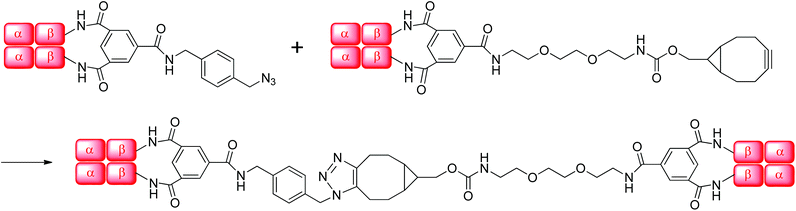 |
| Scheme 3 Cycloaddition of Hb–azide and Hb–cyclooctyne. | |
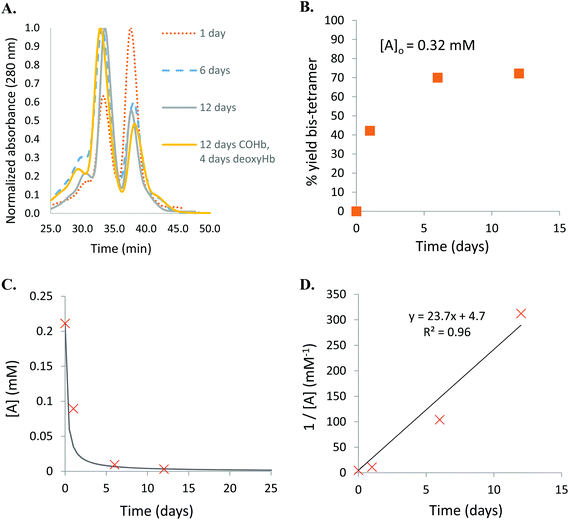 |
| Fig. 3 A. Size-exclusion HPLC trace of the products of the reaction of Hb–cyclooctyne with Hb–azide. The peak at 33 min is due to the ∼128 kDa Hb bis-tetramer and the peak at 38 min is due to the ∼64 kDa cross-linked starting materials. B. Percent yield based on the theoretical maximum. C. Consumption of starting material fit to second order kinetics. D. Linear inverse plot. | |
We assessed the outcome of the SPAAC-based protein-coupling process in comparison to that from CuAAC by coupling a strain-free Hb–alkyne with the Hb–azide in the presence of Cu(I) (Scheme 4). The alkyne-functionalized tether is comparable in length to amine-cyclooctyne so we chose this to lead to effective coupling. A bathophenanthroline ligand (4 eq.), CuSO4 (2 eq.) and ascorbic acid (40 eq.) were added to the protein mixture. This ratio of reagents has previously been shown to be optimal for the coupling of Hb–azides to bis-alkynes.10 However, with the alkyne covalently tethered to Hb, little product formation occurred. After one hour, only a small fraction of the protein present was coupled (Fig. 4). Leaving the reaction mixture longer (greater than one hour) resulted in a significant amount of oxidation of the heme and denaturation of the protein. Methemoglobins were apparent from the deepening colour of the reaction mixture and successive denaturation was confirmed from the observed precipitate. We observed a similar outcome under CuAAC conditions using a Hb–alkyne and a bis–azide.
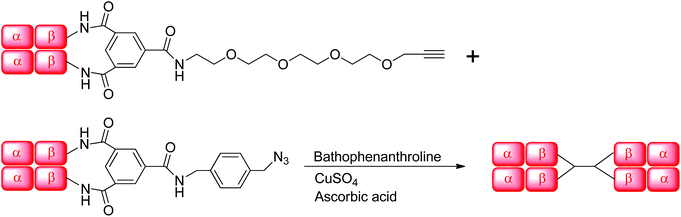 |
| Scheme 4 CuAAC of Hb–alkyne with Hb–azide. | |
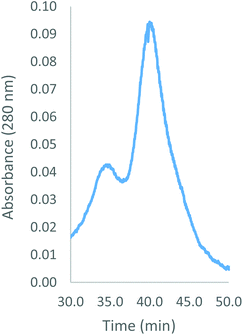 |
| Fig. 4 Size-exclusion HPLC under high salt conditions of the products of CuAAC of Hb–alkyne with Hb–azide. The peak at 34 min is due to the ∼128 kDa Hb bis-tetramer and the peak at 39 min is due to the ∼64 kDa cross-linked reactants. | |
Despite negative results with other combinations (e.g. Hb–alkyne with Hb–azide or bis-azide), Hb–cyclooctyne reacts effectively with a bis-azide (Scheme 5). Approximately 63% bis-tetramer results after incubation at 4 °C for 15 days (Fig. 5). The long incubation does not affect the protein in the absence of copper ion and exclusion of oxygen. Native gel electrophoresis analysis revealed that the species eluting first in the size-exclusion HPLC is the bis-tetramer (Fig. 6). The mass spectrum of the product of the reaction of Hb–cyclooctyne with an excess of bis-azide (see the ESI†) confirms that the bis-azide is capable of reacting with the entire pool of Hb–cyclooctyne. Since every Hb cyclooctyne appendage is accessible to the small molecule bis-azide, then half an equivalent of bis-azide modifies half of the total Hb–cyclooctyne in solution to produce a mixture of approximately 50
:
50 azido Hb to Hb–cyclooctyne. The yield outcome of the bis-tetramer-forming reaction is then analogous to the combination of the singly modified Hb–azide with the Hb–cyclooctyne noted above. Replacing the rigid bis-azide with an extended linkage derived from condensed ethylene glycols (3,6,9,12,15-pentaoxaheptadecane-1,17-diyl bis-azide) did not improve the outcome. The addition of 2.0 or 10.0 eq. of bis-azide resulted in addition of each azide to no more than one Hb-cyclooctyne in contrast to the reaction with a hydrocarbon bis-alkyne.10 The alternative strategy in this case would be inefficient due to the complexity of the reagent.
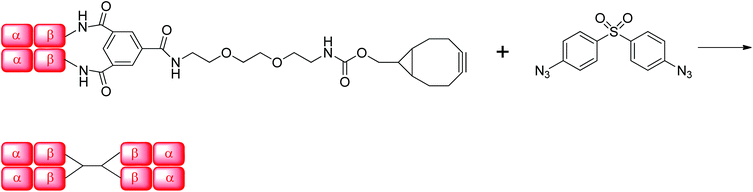 |
| Scheme 5 Preparation of Hb bis-tetramer by SPAAC coupling of Hb–cyclooctyne with a bis-azide. | |
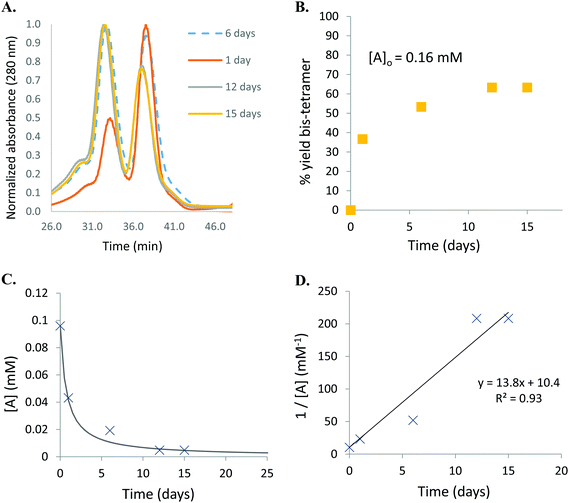 |
| Fig. 5 A. Size-exclusion HPLC trace of the products of the reaction of Hb–cyclooctyne with bis-azide. The peak at 33 min is due to the ∼128 kDa Hb bis-tetramer and the peak at 38 min is due to the ∼64 kDa Hb–cyclooctyne starting material. B. Percent yield based on the theoretical maximum. C. Consumption of starting material fit to second order kinetics. D. Linear inverse plot. | |
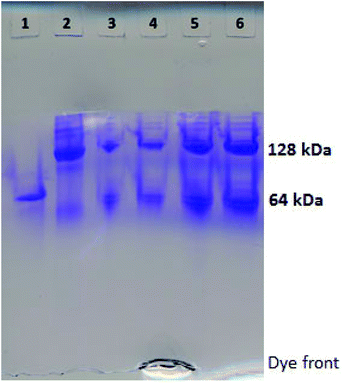 |
| Fig. 6 Native PAGE analysis of the products of the copper-free click reactions after approximately half the starting material is consumed. Lane 1: native Hb; lane 2: pure Hb bis-tetramer reference; lane 3 (also lane 5, at higher sample concentration): products of the reaction of Hb–cyclooctyne with bis-azide; lane 4 (also lane 6, at higher sample concentration): products of the reaction of Hb–cyclooctyne with Hb–azide. | |
The bis-tetramer from SPAAC of Hb–cyclooctyne with bis-azide was separated from the reactants prior to the acquisition of its oxygen-binding curve (Fig. 7). The oxygen affinity of the purified bis-tetramer (P50 = 8.1 ± 0.3 torr) is similar to that of native Hb (P50 = 5 torr) and the cooperativity remains significant in the bis-tetramer (n50 = 2.0 ± 0.1). These oxygen binding properties are comparable to those previously reported for bis-tetramers with structurally analogous features.6,12 Hb properties may be further customized by modifying the internal covalent cross-links31 and/or by adjusting the hybridization of the spacer bridging the two Hb proteins.32
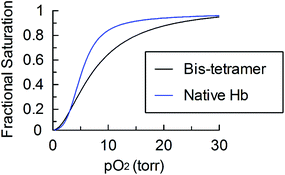 |
| Fig. 7 Oxygen desaturation curve of the purified bis-tetramer from SPAAC of Hb–cyclooctyne with bis-azide compared to that of native Hb. | |
Conclusions
Metal-free strain-promoted azide–alkyne cycloaddition (SPAAC) has been successfully applied for the first time to the coupling cross-linked Hbs. The presence of residual copper from CuAAC is potentially problematic for processes involving heme proteins and the SPAAC process overcomes that limitation. The SPAAC method results in the formation of homogeneous Hb-based bis-tetramers in the highest yields of any process that has been utilized to form members of this class of double proteins (see Table 1 for comparison).
Table 1 Bis-tetramer assembly by SPAAC approach vs. previous methods. Percent yield is the apparent yield calculated from the ratio of bis-tetramer to starting material in the size-exclusion HPLC trace
Approach |
% Yield |
SPAAC |
70 |
CuAAC (Yang et al.12) |
50 |
CuAAC (Foot et al.10) |
20 |
Aminolysis (Lui et al.33) |
40 |
Combining heme proteins under a carbon monoxide atmosphere in a stable non-denaturing environment prevents degradation of the product. This architecture of two coupled Hb tetramers cross-linked between the β-subunits has the desirable physical characteristics of a potential candidate for safe and effective circulatory delivery of oxygen as a supplement to red cells.
Acknowledgements
We thank the Canadian Blood Services for support through an operating grant. S. S. is the recipient of an Ontario Graduate Scholarship.
References
- S. Deroo, F. Stengel, A. Mohammadi, N. Henry, E. Hubin, E. M. Krammer, R. Aebersold and V. Raussens, ACS Chem. Biol., 2015, 10, 1010–1016 CrossRef CAS PubMed.
- R. Kluger and A. Alagic, Bioorg. Chem., 2004, 32, 451–472 CrossRef CAS PubMed.
- T. Schiffner, N. de Val, R. A. Russell, S. W. De Taeye, A. T. De la Pena, G. Ozorowski, H. J. Kim, T. Nieusma, F. Brod, A. Cupo, R. W. Sanders, J. P. Moore, A. B. Ward and Q. J. Sattentau, J. Virol., 2016, 90, 813–828 CrossRef CAS PubMed.
- A. Sinz, C. Arlt, D. Chorev and M. Sharon, Protein Sci., 2015, 24, 1193–1209 CrossRef CAS PubMed.
- A. Alagic, A. Koprianiuk and R. Kluger, J. Am. Chem. Soc., 2005, 127, 8036–8043 CrossRef CAS PubMed.
- F. E. Lui, B. Yu, D. M. Baron, C. Lei, W. M. Zapol and R. Kluger, Transfusion, 2012, 52, 974–982 CrossRef CAS PubMed.
- D. B. Kim-Shapiro, A. N. Schechter and M. T. Gladwin, Arterioscler., Thromb., Vasc. Biol., 2006, 26, 697–705 CrossRef CAS PubMed.
- H. C. Kolb, M. G. Finn and K. B. Sharpless, Angew. Chem., Int. Ed., 2001, 40, 2004–2021 CrossRef CAS.
- E. M. J. Siren, S. Singh and R. Kluger, Org. Biomol. Chem., 2015, 13, 10244–10249 CAS.
- J. S. Foot, F. E. Lui and R. Kluger, Chem. Commun., 2009, 7315–7317 RSC.
- R. Kluger, J. S. Foot and A. A. Vandersteen, Chem. Commun., 2010, 46, 1194–1202 RSC.
- Y. Yang and R. Kluger, Chem. Commun., 2010, 46, 7557–7559 RSC.
- S. Singh, I. S. Dubinsky-Davidchik, Y. Yang and R. Kluger, Org. Biomol. Chem., 2015, 13, 11118 CAS.
- J. M. Rifkind, L. D. Lauer, S. C. Chiang and N. C. Li, Biochemistry, 1976, 15, 5337–5343 CrossRef CAS PubMed.
- V. Hong, S. I. Presolski, C. Ma and M. G. Finn, Angew. Chem., Int. Ed., 2009, 48, 9879–9883 CrossRef CAS PubMed.
- L. M. Gaetke and C. K. Chow, Toxicology, 2003, 189, 147–163 CrossRef CAS PubMed.
- D. Zeng, B. M. Zeglis, J. S. Lewis and C. J. Anderson, J. Nucl. Med., 2013, 54, 829–832 CrossRef CAS PubMed.
- G. R. Abel, Z. A. Calabrese, J. Ayco, J. E. Hein and T. Ye, Bioconjugate Chem., 2016, 27, 698–704 CrossRef CAS PubMed.
- C. Gruttner, K. Muller and J. Teller, IEEE Trans. Magn., 2013, 49, 172–176 CrossRef.
- N. J. Agard, J. A. Prescher and C. R. Bertozzi, J. Am. Chem. Soc., 2004, 126, 15046–15047 CrossRef CAS PubMed.
- P. V. Chang, J. A. Prescher, E. M. Sletten, J. M. Baskin, I. A. Miller, N. J. Agard, A. Lo and C. R. Bertozzi, Proc. Natl. Acad. Sci. U. S. A., 2010, 107, 1821–1826 CrossRef CAS PubMed.
- C. Wendeln, I. Singh, S. Rinnen, C. Schulz, H. F. Arlinghaus, G. A. Burley and B. J. Ravoo, Chem. Sci., 2012, 3, 2479–2484 RSC.
- H. Yang, P. Srivastava, C. Zhang and J. C. Lewis, ChemBioChem, 2014, 15, 223–227 CrossRef CAS PubMed.
- C. Ornelas, J. Broichhagen and M. Weck, J. Am. Chem. Soc., 2010, 132, 3923–3931 CrossRef CAS PubMed.
- S. Schoffelen, J. Beekwilder, M. F. Debets, D. Bosch and J. C. M. V. Hest, Bioconjugate Chem., 2013, 24, 987–996 CrossRef CAS PubMed.
- K. D. Webster, D. Dahhan, C. Frosti, W. Dean, J. B. Chaires and K. W. Olsen, FASEB J., 2016, 30, 825.3 CrossRef PubMed.
- R. Kluger, Y. Song, J. Wodzinska, C. Head, T. S. Fujita and R. T. Jones, J. Am. Chem. Soc., 1992, 114, 9275–9279 CrossRef CAS.
-
C. Arndt, S. Koristka, H. Bartsch and M. Bachmann, in Protein Electrophoresis, ed. B. T. Kurien and R. H. Scofield, Humana Press, 2012, vol. 869, ch. 5, pp. 49–53 Search PubMed.
- R. Kluger and Y. Song, J. Org. Chem., 1994, 59, 733–736 CrossRef CAS.
- J. M. Baskin, J. A. Prescher, S. T. Laughlin, N. J. Agard, P. V. Chang, I. A. Miller, A. Lo, J. A. Codelli and C. R. Bertozzi, Proc. Natl. Acad. Sci. U. S. A., 2007, 104, 16793–16797 CrossRef CAS PubMed.
- M. A. Schumacher, M. M. Dixon, R. Kluger, R. T. Jones and R. G. Brennan, Nature, 1995, 375, 84–87 CrossRef CAS PubMed.
- D. Hu and R. Kluger, Biochemistry, 2008, 47, 12551–12561 CrossRef CAS PubMed.
- F. E. Lui and R. Kluger, Biochemistry, 2009, 48, 11912–11919 CrossRef CAS PubMed.
Footnote |
† Electronic supplementary information (ESI) available: Mass spectra of modified protein subunits and HPLC traces. See DOI: 10.1039/c6ob01817c |
|
This journal is © The Royal Society of Chemistry 2016 |
Click here to see how this site uses Cookies. View our privacy policy here.