DOI:
10.1039/C6NR00272B
(Communication)
Nanoscale, 2016,
8, 7396-7401
Unraveling a generic growth pattern in structure evolution of thiolate-protected gold nanoclusters†
Received
12th January 2016
, Accepted 8th March 2016
First published on 8th March 2016
Abstract
Precise control of the growth of thiolate-protected gold nanoclusters is a prerequisite for their applications in catalysis and bioengineering. Here, we bring to bear a new series of thiolate-protected nanoclusters with a unique growth pattern, i.e., Au20(SR)16, Au28(SR)20, Au36(SR)24, Au44(SR)28, and Au52(SR)32. These nanoclusters can be viewed as resulting from the stepwise addition of a common structural motif [Au8(SR)4]. The highly negative values of the nucleus-independent chemical shift (NICS) in the center of the tetrahedral Au4 units suggest that the overall stabilities of these clusters stem from the local stability of each tetrahedral Au4 unit. Generalization of this growth-pattern rule to large-sized nanoclusters allows us to identify the structures of three new thiolate-protected nanoclusters, namely, Au60(SR)36, Au68(SR)40, and Au76(SR)44. Remarkably, all three large-sized nanoclusters possess relatively large HOMO–LUMO gaps and negative NICS values, suggesting their high chemical stability. Further extension of the growth-pattern rule to the infinitely long nanowire limit results in a one-dimensional (1D) thiolate-protected gold nanowire (RS-AuNW) with a band gap of 0.78 eV. Such a unique growth-pattern rule offers a guide for precise synthesis of a new class of large-sized thiolate-protected gold nanoclusters or even RS-AuNW which, to our knowledge, has not been reported in the literature.
Introduction
Since the first successful crystallization of the thiolate-protected gold nanocluster Au102(SR)44 in 2007,1 research into the structural evolution and structure–property relationship of thiolate-protected gold nanoclusters has attracted considerable attention due to high potential of these nanoclusters for applications in electronics, catalysis and bioengineering.2–7 Significant advancement in structure determination has been made on the basis of X-ray crystallography,1,8–20 single-particle transmission electron microscopy (SP-TEM),21 as well as density-functional theory (DFT) computation22–30 in conjunction with the “divide and protect” formulation.4,31 Although the latter formulation can be very useful in seeking optimal ligand patterns for given gold-core structures, generic growth patterns of the gold nanoclusters are still largely unknown, which hinders the development of large-sized ligand-protected gold nanoclusters for optical and electronic applications.
In this communication, we report a growth-pattern rule that has been revealed based on previously known (via X-ray crystallography) and/or theoretically predicted structures of a series of ligand-protected gold nanoclusters, i.e., Au20(SR)16,25,32 Au28(SR)20,13,40 Au36(SR)24,15 Au44(SR)28,28,33 and Au52(SR)32.12 These clusters can be viewed as structural evolutions from the starting cluster Au20(SR)16via sequential addition of a [Au8(SR)4] motif, i.e., Au20(SR)16 + [Au8(SR)4] → Au28(SR)20 + [Au8(SR)4] → Au36(SR)24 + [Au8(SR)4] → Au44(SR)28 + [Au8(SR)4] → Au52(SR)32. Fig. 1 illustrates the structural evolution of the face-centered-cubic (FCC) type of Au kernels in these clusters via sequential addition of the “boat-like” Au8 motif.
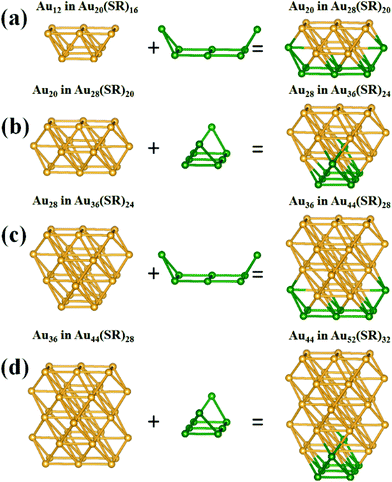 |
| Fig. 1 Au-kernel growth by adding the “boat-like” Au8 motif (olive). (a) Au12 in Au20(SR)16 to Au20 in Au28(SR)20, (b) Au20 in Au28(SR)20 to Au28 in Au36(SR)24, (c) Au28 in Au36(SR)24 to Au36 in Au44(SR)28, (d) Au36 in Au44(SR)28 to Au44 in Au52(SR)32. According to the deduction of Zeng et al.,33 the structures of these nanoclusters can be characterized as Au12[Au2(SR)3]4(SR)4, Au20[Au2(SR)3]4(SR)8/Au20[Au(SR)2]2[Au2(SR)3]2(SR)8, Au28[Au2(SR)3]4(SR)12, Au36[Au2(SR)3]4(SR)16, and Au44[Au2(SR)3]4(SR)20, respectively. Next, the Au-kernels can be derived by removing the [Au2(SR)3] staple motifs and the bridging thiolates (–SR–). | |
Computational methods
All clusters were optimized using the DFT method implemented in the Dmol3 7.0 code.34,35 To this end, the generalized gradient approximation in the Perdew–Burke–Ernzerhof (PBE)36 form was employed together with the double numeric polarized (DNP) basis set and the semi-core pseudopotential. In all our computations, the R group in the ligands is simplified as a methyl group or hydrogen atom. All the optmized structures of the nanoclusters are presented in ESI Fig. S1.† On basis of the optmized structures (R = hydrogen atom), nucleus-independent chemical shift (NICS) analysis was performed to examine the aromaticity of the clusters using the B3LYP functional37,38 with LANL2DZ and 6-31G* basis set implemented in the Gaussian 09 package.39
Results and discussion
As shown in Table 1, the atomic structures of Au28(SR)20,13 Au36(SR)24,15 and Au52(SR)32
20 nanoclusters have been determined via X-ray crystallography. Note that Au20(SR)16 may have two isomeric structures. The theoretically predicted structure25 (see Fig. 1(a)) can well reproduce the optical absorption spectrum of Au20(PET)16 (PET = SCH2CH2Ph),33 and it has a distinct Au kernel of the crystallized Au20(TBBT)16 (TBBT = SPh-t-Bu) cluster.8 However, considering the close total energy of these two structures (their energy difference is 0.3 eV with capped methyl groups for simplicity), one of the two isomers is likely the same as the theoretically predicted structure. A similar situation was reported in the cases of Au40(o-MBT)24
43 (o-MBT = ortho-methylbenzenethiol) and Au40(PET)24.44 The structure prediction of Au44(SR)28
28,33 was based on the structural rules derived from the crystallization of Au28(SR)20 and Au36(SR)24, i.e., Au28(SR)20 + [Au8(SR)4] → Au36(SR)24 (Fig. 1(b)) + [Au8(SR)4] → Au44(SR)28 (Fig. 1(c)). The excellent agreement between experimental and computed optical absorption spectra of Au44(SR)28 validates the predicted structure of Au44(SR)28, which is solely based on the growth-pattern rule derived from Au28(SR)20 and Au36(SR)24. Additional evidence comes from the crystallization of the Au52(SR)32 nanocluster,20 whose structure can be viewed as adding the [Au8(SR)4] motif to the predicted structure of Au44(SR)28 (Fig. 1(d)). It should be noted that the recently reported Au28(S-c-C6H11)20 (where -c-C6H11 = cyclohexyl)40 cluster exhibits a similar Au20 kernel structure to crystallized Au28(TBBT)20 (ESI Fig. S2†). Therefore, the two crystallized structures of Au28(SR)20 can be evolved from Au20(SR)16 (Fig. 1(a)). Besides the different surface-protecting ligands, the two isomeric structures have different staple motifs.
Table 1 A summary of literature results for a series of ligand-protected gold nanoclusters from Au20(SR)16 to Au76(SR)44. √: structure determined from X-ray crystallography; p: theoretical prediction; \: synthesized clusters without crystallization; ?: structure unknown; ‡: difference in crystallization, prediction not yet confirmed
According to the “divide and protect” formulation,45 Au20(SR)16, Au28(SR)20, Au36(SR)24, Au44(SR)28, and Au52(SR)32 can be divided into Au8[Au3(SR)4]4, Au14[Au2(SR)3]4[Au3(SR)4]2/Au14[Au(SR)2]2[Au2(SR)3]4, Au20[Au2(SR)3]8, Au26[Au(SR)2]2[Au2(SR)3]8, and Au32[Au(SR)2]4[Au2(SR)3]8, respectively. The Au8 in Au20(SR)16, Au14 in Au28(SR)20, Au20 in Au36(SR)24, Au26 in Au44(SR)28, and Au32 in Au52(SR)32, which are comprised of tetrahedral Au4 units, can be derived by removing the [Au(SR)2], [Au2(SR)3], and [Au3(SR)4] staple motifs, as shown in Fig. 2. It is known that NICS values have been commonly used as an index to measure the local aromaticity of fullerene cages46 or small metal clusters.47 In addition, NICS analyses have been applied successfully to evaluate the stabilities of gold fullerene structures, such as Au32
48 and Au42.49 Recently, NICS analyses of the Au20(SR)16 cluster were performed to support the concept of a superatom-network (SAN);50 a theory to explain the stability of thiolate-protected gold nanoclusters. Thus, it is sensible to examine the stabilities of Au20(SR)16, Au28(SR)20, Au36(SR)24, Au44(SR)28, and Au52(SR)32 based on the computed NICS values corresponding to the center of the tetrahedral Au4 units of these clusters. It can be seen that the absolute NICS values (see Table 2) at the centers of the tetrahedral Au4 units are large; larger than those at the centers of the total structures. The NICS results suggest notable aromaticity in the tetrahedral Au4 units. As such, the overall stabilities of Au20(SR)16, Au28(SR)20, Au36(SR)24, Au44(SR)28, and Au52(SR)32 likely stem from the local stability of each tetrahedral Au4 unit.
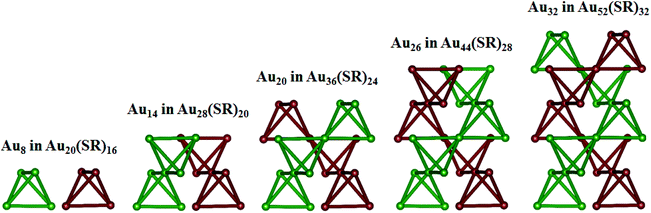 |
| Fig. 2 The structures of Au8 in Au20(SR)16, Au14 in Au28(SR)20, Au20 in Au36(SR)24, Au26 in Au44(SR)28, and Au32 in Au52(SR)32. Au atoms are both olive and wine. | |
Table 2 Computed nucleus-independent chemical shift (NICS) values of Au20(SR)16, Au28(SR)20, Au36(SR)24, Au44(SR)28, and Au52(SR)32. “0” denotes the NICS values at the centers of the total structures. “1–10” denote the NICS values at the centers of tetrahedral Au4 units of these clusters (ESI Fig. S3)
|
0 |
1 |
2 |
3 |
4 |
5 |
6 |
7 |
8 |
9 |
10 |
Au20(SR)16 |
−7.5 |
−28.2 |
−28.7 |
|
|
|
|
|
|
|
|
Au28(SR)20 |
−12.4 |
−25.3 |
−26.3 |
−25.2 |
−26.5 |
|
|
|
|
|
|
Au36(SR)24 |
−10.8 |
−24.5 |
−26.2 |
−23.4 |
−25.1 |
−24.2 |
−25.7 |
|
|
|
|
Au44(SR)28 |
−17.3 |
−25.6 |
−23.5 |
−23.5 |
−25.6 |
−25.6 |
−23.8 |
−23.5 |
−25.6 |
|
|
Au52(SR)32 |
−12.7 |
−25.9 |
−25.1 |
−24.2 |
−22.1 |
−25.5 |
−26.0 |
−23.1 |
−23.0 |
−24.7 |
−25.7 |
The unique growth-pattern rule derived among the series of clusters, Au20(SR)16, Au28(SR)20, Au36(SR)24, Au44(SR)28 and Au52(SR)32, suggests the possible existence of larger-sized clusters through continuously adding the motif [Au8(SR)4], e.g., Au52(SR)32 + [Au8(SR)4] → Au60(SR)36 + [Au8(SR)4] → Au68(SR)40 + [Au8(SR)4] → Au76(SR)44, where the newly created Au60(SR)36, Au68(SR)40, and Au76(SR)44 all possess the FCC-type Au-kernels (Fig. 3). Fig. 4 presents the computed HOMO–LUMO gaps of the optimized nanoclusters from the small-sized Au20(SR)16 to large-sized Au76(SR)44, as well as the experimentally measured optical gaps of Au20(SR)16, Au28(SR)20, Au36(SR)24, and Au44(SR)28. The computed gaps reproduce the experimental gaps quite well except for Au28(SR)20. Nevertheless, the computed gap of Au28(SR)20 is consistent with a previous theoretical study.14 The HOMO–LUMO gaps of Au60(SR)36, Au68(SR)40, and Au76(SR)44 are all greater than 1.0 eV, comparable to those of Au64(SC6H11)32
51 and Au67(PET)35.52 Double-helix structures made of tetrahedral Au4 units can be seen in the three new structures (ESI Fig. S4†), and are also present in Au20(SR)16, Au28(SR)20, Au36(SR)24, Au44(SR)28 and Au52(SR)32 clusters. Furthermore, the NICS analyses (ESI Table S1 and Fig. S6†) also show that the overall stabilities of Au60(SR)36, Au68(SR)40, and Au76(SR)44 are likely due to the local stability of each tetrahedral Au4 unit. The large HOMO–LUMO gaps and the negative NICS values suggest high stability of the newly predicted structures.
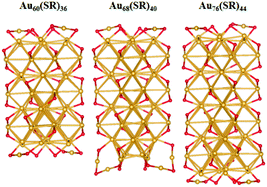 |
| Fig. 3 The optimized structures of Au60(SR)36, Au68(SR)40, and Au76(SR)44, where the methyl groups are omitted for clarity. Au and S atoms are in gold and red, respectively. | |
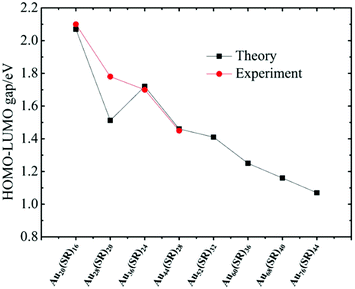 |
| Fig. 4 The computed HOMO–LUMO gaps of the optimized nanoclusters from small-sized Au20(SR)16 to large-sized Au76(SR)44, and the measured optical gaps. | |
It should be noted that the Au76(4-MEBA)44 (4-MEBA = 4-(2-mercaptoethyl)benzoic acid) nanocluster has been synthesized recently by Takano et al.53 Although Au76(4-MEBA)44 has the same number of Au atoms and ligands as Au76(SR)44, a comparison of the computed and experimental X-ray diffraction (XRD) and optical absorption spectra suggests that the two clusters may have different structures in their Au-kernels (ESI Fig. S5†). It is known that surface-protecting thiolates can have significant effects on the structures of gold nanoclusters even with the same number of Au and S atoms. For example, the marked differences in their absorption spectra indicate that Au40(o-MBT)24
43 and Au40(PET)24
44 have different structures, and so do Au20(PET)16
32 and Au20(TBBT)16,8 as well as Au28(TBBT)20
13 and Au28(S-c-C6H11)20.40 Moreover, even with the same ligands, different Au38(PET)24 isomers have been detected.15,54
Lastly, if the growth-pattern rule is extended to the infinitely-long nanowire limit by repeatedly adding [Au8(SR)4] units in one direction, the thiolate-protected gold nanowire (RS-AuNW) can be obtained, as shown in Fig. 5(a) and (b). The present RS-AuNW also exhibits a double-helix structure made of tetrahedral Au4 units, which is very different from that of previously proposed vertex- and face-sharing icosahedral thiolated Au nanowires55 and crystallized [Au25(SBu)180]n nanowires.56Fig. 5(c) shows the computed total density of state (DOS) of the present RS-AuNW, which shows an electronic band gap of 0.78 eV, suggesting that the present RS-AuNW is semiconducting. The vertex-sharing thiolated gold nanowire can be made either semiconducting or metallic by tuning the charge. The face-sharing nanowire is always metallic. The nonmagnetic ground state of [Au25(SBu)180]n has a band gap of 0.12 eV, suggesting that [Au25(SBu)180]n could behave as a narrow-gap semiconductor. It is also found that the valence band of the present RS-AuNW is mainly contributed to by the Au(5d), S(3p) Au(6s), and Au(6p) atomic orbitals, while the conduction band is mainly due to the Au(6sp) atomic orbitals.
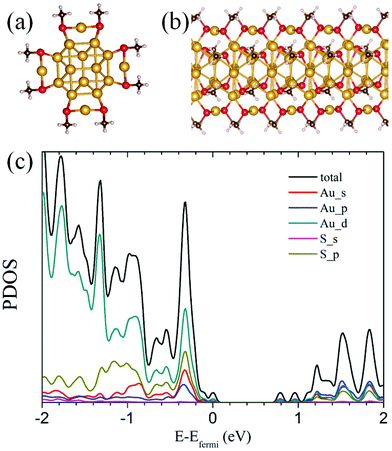 |
| Fig. 5 The proposed structure of the thiolate-protected gold nanowire: (a) viewed along the wire; (b) side view. Au, S, C, and H atoms are in gold, red, dark gray, and white, respectively. (c) Computed projected density of state (PDOS) of the thiolate-protected gold nanowire. Au_s, Au_p, and Au_d denote the s, p, and d orbitals of Au atoms, respectively. S_s and S_p denote the s and p orbitals of S atoms, respectively. | |
Conclusions
In conclusion, a generic growth-pattern rule is identified based on the series of nanoclusters Au20(SR)16, Au28(SR)20, Au36(SR)24, Au44(SR)28, and Au52(SR)32, which can be viewed as the sequential addition of [Au8(SR)4] units. The large negative nucleus-independent chemical shift (NICS) values in the centers of the tetrahedral Au4 units indicate that the integral stabilities of these clusters are determined by the local stability of each tetrahedral Au4 unit. Extension of the rule to larger-sized nanoclusters than the state-of-the-art gives rise to new structures of nanoclusters such as Au60(SR)36, Au68(SR)40, and Au76(SR)44. All three large-sized nanoclusters exhibit relatively large HOMO–LUMO gaps and negative NICS values, suggesting their high chemical stability. It is also found that the computed XRD and optical absorption spectra of Au76(SR)44 are not the same as those of Au76(4-MEBA)44 from experiments, suggesting the two nanoclusters may have different Au-kernel structures. Finally, extension of the growth-pattern rule to the infinitely long nanowire limit results in a 1D RS-AuNW with a band gap of 0.78 eV. The unique growth-pattern rule offers a guide for future synthesis of a new class of large-sized thiolate-protected gold nanoclusters or even RS-AuNW that has not been reported in the literature.
Acknowledgements
W.W.X. is supported by the China postdoctoral science foundation project (Y419022011, Y519031011), and National Natural Science Foundation of China (11504396). Y.G. is supported by start-up funding from the Shanghai Institute of Applied Physics, Chinese Academy of Sciences (Y290011011), National Natural Science Foundation of China (21273268, 11574340), “Hundred People Project” from Chinese Academy of Sciences, “Pu-jiang Rencai Project” from Science and Technology Commission of Shanghai Municipality (13PJ1410400), and CAS-Shanghai Science Research Center (CAS-SSRC-YJ-2015-01). The computational resources utilized in this research were provided by Shanghai Supercomputer Center, National Supercomputer Centers in Tianjin and Shenzhen, and special program for applied research on super-computation of the NSFC-Guangdong joint fund (the second phase). X.C.Z. is supported by the USTC Qian-ren B (1000-Talents Program B) fund for summer research.
References
- P. D. Jadzinsky, G. Calero, C. J. Ackerson, D. A. Bushnell and R. D. Kornberg, Science, 2007, 318, 430–433 CrossRef CAS PubMed.
- R. Jin, Nanoscale, 2010, 2, 343–362 RSC.
- H. Häkkinen, Nat. Chem., 2012, 4, 443–455 CrossRef PubMed.
- Y. Pei and X. C. Zeng, Nanoscale, 2012, 4, 4054–4072 RSC.
- H. Qian, M. Zhu, Z. Wu and R. Jin, Acc. Chem. Res., 2012, 45, 1470–1479 CrossRef CAS PubMed.
- R. Jin, Nanoscale, 2015, 7, 1549–1565 RSC.
- R. W. Murray, Chem. Rev., 2008, 108, 2688–2720 CrossRef CAS PubMed.
- A. Das, C. Liu, H. Y. Byun, K. Nobusada, S. Zhao, N. Rosi and R. Jin, Angew. Chem., Int. Ed., 2015, 54, 3140–3144 CrossRef CAS PubMed.
- C. Zeng, C. Liu, Y. Chen, N. L. Rosi and R. Jin, J. Am. Chem. Soc., 2014, 136, 11922–11925 CrossRef CAS PubMed.
- D. Crasto, G. Barcaro, M. Stener, L. Sementa, A. Fortunelli and A. Dass, J. Am. Chem. Soc., 2014, 136, 14933–14940 CrossRef CAS PubMed.
- M. Zhu, C. M. Aikens, F. J. Hollander, G. C. Schatz and R. Jin, J. Am. Chem. Soc., 2008, 130, 5883–5885 CrossRef CAS PubMed.
- M. W. Heaven, A. Dass, P. S. White, K. M. Holt and R. W. Murray, J. Am. Chem. Soc., 2008, 130, 3754–3755 CrossRef CAS PubMed.
- C. Zeng, T. Li, A. Das, N. L. Rosi and R. Jin, J. Am. Chem. Soc., 2013, 135, 10011–10013 CrossRef CAS PubMed.
- D. Crasto, S. Malola, G. Brosofsky, A. Dass and H. Häkkinen, J. Am. Chem. Soc., 2014, 136, 5000–5005 CrossRef CAS PubMed.
- C. Zeng, H. Qian, T. Li, G. Li, N. L. Rosi, B. Yoon, R. N. Barnett, R. L. Whetten, U. Landman and R. Jin, Angew. Chem., Int. Ed., 2012, 51, 13114–13118 CrossRef CAS PubMed.
- H. Qian, W. T. Eckenhoff, Y. Zhu, T. Pintauer and R. Jin, J. Am. Chem. Soc., 2010, 132, 8280–8281 CrossRef CAS PubMed.
- Y. Chen, C. Zeng, C. Liu, K. Kirschbaum, C. Gayathri, R. R. Gil, N. L. Rosi and R. Jin, J. Am. Chem. Soc., 2015, 137, 10076–10079 CrossRef CAS PubMed.
- A. Dass, S. Theivendran, P. R. Nimmala, C. Kumara, V. R. Jupally, A. Fortunelli, L. Sementa, G. Barcaro, X. Zuo and B. C. Noll, J. Am. Chem. Soc., 2015, 137, 4610–4613 CrossRef CAS PubMed.
- C. Zeng, Y. Chen, K. Kirschbaum, K. Appavoo, M. Y. Sfeir and R. Jin, Sci. Adv., 2015, 1, e1500045 Search PubMed.
- C. Zeng, Y. Chen, C. Liu, K. Nobusada, N. L. Rosi and R. Jin, Sci. Adv., 2015, 1, e1500425 Search PubMed.
- M. Azubel, J. Koivisto, S. Malola, D. Bushnell, G. L. Hura, A. L. Koh, H. Tsunoyama, T. Tsukuda, M. Pettersson, H. Häkkinen and R. D. Kornberg, Science, 2014, 345, 909–912 CrossRef CAS PubMed.
- J. Akola, M. Walter, R. L. Whetten, H. Häkkinen and H. Grönbeck, J. Am. Chem. Soc., 2008, 130, 3756–3757 CrossRef CAS PubMed.
- W. W. Xu, Y. Gao and X. C. Zeng, Sci. Adv., 2015, 1, e1400211 Search PubMed.
- W. W. Xu and Y. Gao, J. Phys. Chem. C, 2015, 119, 14224–14229 CAS.
- Y. Pei, Y. Gao, N. Shao and X. C. Zeng, J. Am. Chem. Soc., 2009, 131, 13619–13621 CrossRef CAS PubMed.
- Y. Pei, R. Pal, C. Liu, Y. Gao, Z. Zhang and X. C. Zeng, J. Am. Chem. Soc., 2012, 134, 3015–3024 CrossRef CAS PubMed.
- Y. Pei, Y. Gao and X. C. Zeng, J. Am. Chem. Soc., 2008, 130, 7830–7832 CrossRef CAS PubMed.
- Y. Pei, S. S. Lin, J. Su and C. Liu, J. Am. Chem. Soc., 2013, 135, 19060–19063 CrossRef CAS PubMed.
- Y. Pei, J. Tang, X. Tang, Y. Huang and X. C. Zeng, J. Phys. Chem. Lett., 2015, 6, 1390–1395 CrossRef CAS PubMed.
- D. Jiang, S. H. Overbury and S. Dai, J. Am. Chem. Soc., 2013, 135, 8786–8789 CrossRef CAS PubMed.
- H. Häkkinen, M. Walter and H. Gronbeck, J. Phys. Chem. B, 2006, 110, 9927–9931 CrossRef PubMed.
- M. Zhu, H. Qian and R. Jin, J. Am. Chem. Soc., 2009, 131, 7220–7221 CrossRef CAS PubMed.
- C. Zeng, Y. Chen, G. Li and R. Jin, Chem. Commun., 2014, 50, 55–57 RSC.
- B. Delley, J. Chem. Phys., 1990, 92, 508–517 CrossRef CAS.
- B. Delley, J. Chem. Phys., 2003, 113, 7756–7764 CrossRef . Dmol3 is available from Accelrys.
- J. P. Perdew, K. Burke and M. Ernzerhof, Phys. Rev. Lett., 1996, 77, 3865–3868 CrossRef CAS PubMed.
- A. D. Becke, Phys. Rev. A, 1988, 38, 3098 CrossRef CAS.
- A. Lee, W. Yang and R. G. Parr, Phys. Rev. B: Condens. Matter, 1988, 37, 785–789 CrossRef.
-
M. J. Frisch, G. W. Trucks, H. B. Schlegel, G. E. Scuseria, M. A. Robb, J. R. Cheeseman, V. G. Zakrzewski, J. A. Montgomery Jr., R. E. Stratmann, J. C. Burant, S. Dapprich, J. M. Millam, A. D. Daniels, K. N. Kudin, M. C. Strain, O. Farkas, J. Tomasi, V. Barone, M. Cossi, R. Cammi, B. Mennucci, C. Pomelli, C. Adamo, S. Clifford, J. Ochterski, G. A. Petersson, P. Y. Ayala, Q. Cui, K. Morokuma, D. K. Malick, A. D. Rabuck, K. Raghavachari, J. B. Foresman, J. Cioslowski, J. V. Ortiz, B. B. Stefanov, G. Liu, A. Liashenko, P. Piskorz, I. Komaromi, R. Gomperts, R. L. Martin, D. J. Fox, T. Keith, M. A. Al-Laham, C. Y. Peng, A. Nanayakkara, C. Gonzalez, M. Challacombe, P. M. W. Gill, B. Johnson, W. Chen, M. W. Wong, J. L. Andres, C. Gonzalez, M. Head-Gordon, E. S. Replogle and J. A. Pople, Gaussian09 (Revision A.02), Gaussian, Inc., Wallingford CT, 2009 Search PubMed.
- Y. Chen, C. Liu, Q. Tang, C. Zeng, T. Higaki, A. Das, D. Jiang, N. L. Rosi and R. Jin, J. Am. Chem. Soc., 2016, 138, 1482–1485 CrossRef CAS PubMed.
- S. K. Knoppe, S. Malola, T. Burgi and H. Häkkinen, J. Phys. Chem. A, 2013, 117, 10526–10533 CrossRef CAS PubMed.
- D. Jiang, M. Walter and J. Akola, J. Phys. Chem. C, 2010, 114, 15883–15889 CAS.
- Y. Chen, C. Zeng, D. R. Kauffman and R. Jin, Nano Lett., 2015, 15, 3603–3609 CrossRef CAS PubMed.
- H. Qian, Y. Zhu and R. Jin, J. Am. Chem. Soc., 2010, 132, 4583–4585 CrossRef CAS PubMed.
- H. Häkkinen, M. Walter and H. Gronbeck, J. Phys. Chem. B, 2006, 110, 9927–9931 CrossRef PubMed.
- A. Hirsch, Z. Chen and H. Jiao, Angew. Chem., Int. Ed., 2000, 39, 3915–3917 CrossRef CAS.
- I. Boldyrev and L. S. Wang, Chem. Rev., 2005, 105, 3716–3757 CrossRef PubMed.
- M. P. Johansson, D. Sundholm and J. Vaara, Angew. Chem., Int. Ed., 2004, 43, 2678–2681 CrossRef CAS.
- Y. Gao and X. C. Zeng, J. Am. Chem. Soc., 2005, 127, 3698–3699 CrossRef CAS PubMed.
- L. Cheng, Y. Yuan, X. Zhang and J. Yang, Angew. Chem., Int. Ed., 2013, 52, 9035–9039 CrossRef CAS PubMed.
- C. Zeng, Y. Chen, G. Li and R. Jin, Chem. Mater., 2014, 26, 2635–2641 CrossRef CAS.
- P. R. Nimmala, B. Yoon, R. L. Whetten, U. Landman and A. Dass, J. Phys. Chem. A, 2013, 117, 504–517 CrossRef CAS PubMed.
- S. Takano, S. Yamazoe, K. Koyasu and T. Tsukuda, J. Am. Chem. Soc., 2015, 137, 7027–7030 CrossRef CAS PubMed.
- S. Tian, Y. Li, M. Li, J. Yuan, J. Yang, Z. Wu and R. Jin, Nat. Commun., 2015, 6, 8667 CrossRef CAS PubMed.
- D. Jiang, K. Nobusada, W. Luo and R. L. Whetten, ACS Nano, 2009, 3, 2351–2357 CrossRef CAS PubMed.
- M. D. Nardi, S. Antonello, D. Jiang, F. Pan, K. Rissanen, M. Ruzzi, A. Venzo, A. Zoleo and F. Maran, ACS Nano, 2014, 8, 8505–8512 CrossRef PubMed.
Footnote |
† Electronic supplementary information (ESI) available. See DOI: 10.1039/c6nr00272b |
|
This journal is © The Royal Society of Chemistry 2016 |