DOI:
10.1039/C5MD00332F
(Concise Article)
Med. Chem. Commun., 2016,
7, 132-140
Design and synthesis of peptide inhibitor conjugates as probes of the Cytochrome P450s from glycopeptide antibiotic biosynthesis†
Received
7th August 2015
, Accepted 24th September 2015
First published on 25th September 2015
Abstract
The glycopeptide antibiotics are important clinical antibiotics that are currently employed against serious Gram-positive bacterial infections. Their chemical complexity means that their production is reliant upon the natural biosynthesis pathway, of which the key steps are the synthesis and side chain cyclisation of the peptide backbone. These processes rely upon a non-ribosomal peptide synthetase (NRPS) and several Cytochrome P450 enzymes that interact with the NRPS-bound peptide to generate the active aglycone. These P450 catalysts, known as Oxy enzymes, are crucial for the biosynthesis and yet the nature of their interactions with substrate peptides remains poorly understood. In this work, we have developed a range of synthetic inhibitor peptide substrates conjugated to an extended phosphopantetheine linker mimic and apply these to the characterisation of the first P450 from vancomycin biosynthesis, OxyBvan. These compounds display improved solubility and binding properties in comparison to isolated GPA precursor peptides and provide insights into the binding of such peptides to the P450 active site. These probes are applicable to the characterisation of other P450s from related biosynthetic machineries and are promising candidates for co-crystallisation with the Oxy enzymes.
Introduction
The Cytochrome P450s are a superfamily of heme-containing monooxygenases widely found in nature.1 These enzymes, which contain the heme ligated via a thiolate ligand in the active site, are capable of catalysing many different oxidative transformations that include, amongst others, regio- and stereoselective hydroxylation of non-activated C–H bonds, aromatic hydroxylation, epoxidation, heteroatom oxidation and aromatic cross coupling.2 The nature of the thiolate ligand combined with careful arrangement of the enzyme active site allows P450s to access reactive intermediates that are capable of performing these transformations.1 What makes these enzymes even more impressive from a chemical perspective is that this large range of chemical reactions can be specifically targeted to a wide range of different substrates, a feature that is particularly important during the biosynthesis of natural products.2
Cytochrome P450s that utilise carrier protein-bound substrates
Within the Cytochrome P450 superfamily, a subgroup of P450s involved in biosynthesis accept their substrates bound to other proteins rather than free in solution;3,4 typically, these carrier proteins (CP) are found within non-ribosomal peptide synthetases (NRPSs) or polyketide synthases (PKSs), biosynthetic mega-enzyme machineries responsible for formation of a wide range of secondary metabolites.5,6 P450s are commonly involved in the biosynthesis of both polyketides and non-ribosomal peptides due to their ability to selectively perform difficult oxidations of complex compounds: however, the group of P450s that act on carrier protein bound substrates is smaller and is largely confined to NRPS-systems.4 The relatively small numbers of P450s that require CP-bound substrates is offset by the importance of the natural products that involve P450s in their biosynthesis: in the case of aminoacyl-CP oxidation this includes both the aminocoumarin antibiotics and the glycopeptide antibiotics, whilst in the case of phenolic and aryl crosslinking this again includes the glycopeptide antibiotics (GPAs, Fig. 1) and arylomycin.7,8
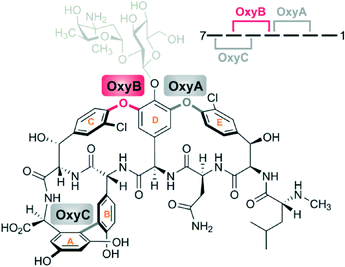 |
| Fig. 1 Structure of vancomycin indicating the sites of P450-catalysed side chain crosslinking; OxyBvan (indicated in red) acts first to install the aglycone C-O-D ring. | |
The challenging nature of these P450-catalysed phenolic/aryl crosslinking transformations makes understanding the mechanism of these enzymes important not only from the standpoint of understanding their biosynthesis – which in the case of the glycopeptide antibiotics remains the only viable method of production – but also crucial to future redesign of these natural products and their potential diversification through combined synthetic and biochemical approaches.9,10
Structural characterisation of CP-binding Cytochrome P450s
Whilst many P450s have been structurally characterised11 – including for those that interact with carrier proteins12–19 – the characterisation of P450s in a carrier protein (and thus substrate) bound state is limited to the P450BioI acyl-ACP system from the biotin biosynthesis pathway employed by certain species of Bacillus.17,20 P450BioI performs the oxidative cleavage of fatty acids specifically between C7 and C8 of the fatty acid chain, and the structural characterisation of this complex was crucial to determining how this enzyme is able to perform this reaction and why the carrier protein is required to maintain P450 selectivity.17,20 However, the ability to isolate this CP/P450 complex and eventually structurally characterise it was due in no small part to the unusually tight interaction between these two proteins, mediated through a combination of electrostatic, hydrogen bonding and hydrophobic interactions.17 The carrier proteins from PKS/NRPS systems typically do not share the ability to form such tight protein–protein interactions, which is necessitated by the requirement for these CP-domains to interact with multiple catalytic domains in both cis and trans to allow biosynthesis of polyketides or peptides to occur.21,22 In the case of the P450 (Oxy) enzymes involved in GPA biosynthesis we have recently shown that their recruitment to the peptidyl-PCP (Peptidyl Carrier Protein) substrate is mediated through a separate recruitment domain known as the X-domain, whilst P450 activity against isolated carrier proteins is typically limited.23 This complicates the investigation of such interactions and reactions steps tremendously, and has necessitated the development of sophisticated approaches to investigate such systems.
Due to the difficulty of obtaining carrier protein/P450 complexes for structural analysis, we previously developed and exploited the use of an inhibitor approach to enhance the binding of the carrier protein substrate to the P450:13 through this approach, the isolation of a carrier protein/P450 complex from skyllamycin biosynthesis was enabled.24 Here, it was necessary to use inhibitors covalently bound to the phosphopantetheine linker of the PCP. In such systems, shorter substrate-like mimics were not accepted as substrates and displayed atypical binding to these P450s.25 From a structural perspective this is likely due to the optimisation of the P450 interaction interface for carrier protein binding, which itself is necessitated by the need to avoid the inherent risk of interfering with primary metabolism due to uncontrolled amino acid hydroxylation.16
Glycopeptide antibiotic biosynthetic Cytochrome P450s
In the case of the glycopeptide antibiotic biosynthetic P450s, it has been demonstrated that shorter substrate mimics, including peptidyl-CoA adducts, N-acetylcysteamine (SNAc) derivatives and free peptides are accepted as in vitro substrates, albeit at a low level, by the first oxygenase from vancomycin biosynthesis, OxyBvan.26 Activity against such small molecule substrates raises the hope of using these to obtain a substrate bound Oxy structure: however, to date no such substrate-bound structure has been reported.
Given the importance of these P450-catalysed transformations and the current lack of information concerning the binding mode of the peptides within the Oxy active site we decided to design and synthesise a series of glycopeptide linker peptide substrate mimics based around the concept of P450 inhibitors.13 In addition to the potential improvement in complex stability as was demonstrated for P450sky the use of nitrogen-containing amino acids within the peptide would provide information about the approach of these amino acid side chains towards the heme of the P450, which could be interrogated by observing the change in spectral signal of the heme iron upon coordination of nitrogen causing a so-called type-II spectral shift (demonstrating a spectral maximum at 425 nm and minimum at 410 nm during binding). For these substrates, we decided to synthesise peptide conjugates bound to 3-acetamido-N-(2-aminoethyl)propanamide 1 – a phosphopantetheine (PPant)-linker mimic – rather than a CoA or SNAc conjugate (Fig. 2). As this PPant linker mimic would be connected via an amide bond with the peptides, these conjugates would be hydrolytically stable whilst providing the majority of the structure of the natural PPant linker to allow potential interactions with the P450 to be maintained. An additional benefit of using 1 is the increase in polarity of such peptide conjugates over the unmodified peptides or their SNAc derivatives: given the highly non-polar nature of GPA precursor peptides, we also anticipated that the linker peptides would show improved properties in binding assays, particularly in relation to the amount of scattered light typically observed due to aggregation of the non-polar peptides at high concentrations.
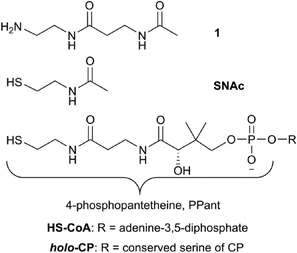 |
| Fig. 2 Structure of the 4-phosphopantetheine post-translational modification of carrier proteins and small molecule mimics of this moiety: these include the SNAc group as well as the stabilised linker 1 discussed in this work. | |
Results and discussion
Peptide synthesis utilising hydrazinobenzoyl resin
In order to design an efficient route for the synthesis of these inhibitors, we modified our reported solid phase peptide synthesis route based upon Fmoc-chemistry27,28 to utilise hydrazinobenzoyl resin29 rather than the standard thioester route using Dawson-resin (Fig. 3).30 Hydrazinobenzoyl resin (2) offers the ability to isolate peptide esters and amides directly following oxidative cleavage of the peptide from the resin: the oxidation of the hydrazine linker generates a highly reactive aryl diazene group (4) that is readily displaced as nitrogen in the presence of a nucleophile.29,31 Thus, we synthesised our hexa- and heptapeptide probes 5–14 on hydrazinobenzoyl resin and then added the amine-containing PPant linker mimic 1 during oxidative workup of the resin to generate the desired peptide linker inhibitors (Fig. 3 and 5); due to the relatively high polarity of the linker amine, it was necessary to alter the typical workup solvent from dichloromethane to DMF and to increase the reaction time up to four hours in order to achieve efficient synthesis of the desired linker peptides.
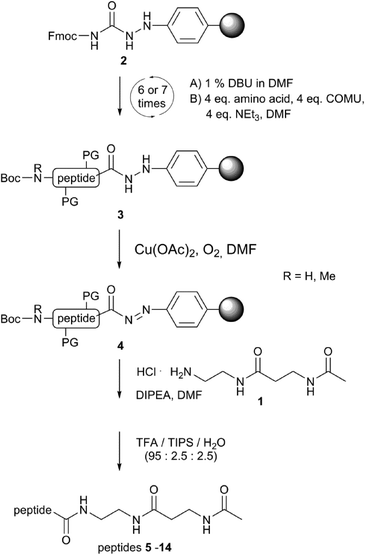 |
| Fig. 3 Solid phase synthesis route generating the peptide linker compounds 5–14 using hydrazinobenzoyl resin and displacement with linker amine 1 (PG: Asn – trityl; Tyr – t-butyl; His – trityl; Lys – Boc). | |
Initial compound design and assay development
Our initial targets for synthesis were the hexa- and heptapeptide conjugates (5–7) based upon the structure of vancomycin (Fig. 5).8 In order to assess binding of these substrates, we examined the effect that these substrates had on the heme absorbance of OxyBvan (419 nm heme soret peak). Whilst the typical P450 type-I spectra, which is commonly caused by the displacement of the water molecule bound to the heme iron upon substrate binding (demonstrating a spectral maximum at 390 nm and minimum at 425 nm during binding) has been observed for some peptide/protein carrier combinations, OxyBvan also exhibits an unusual spectral signature for substrate binding with certain substrates that displays a spectral maximum at 410 nm and small minimum at 435 nm (here termed type-III binding).26,32,33 The cause of this unusual binding signature is unclear, although this binding mode has been demonstrated to lead to product formation and is thus also relevant to catalysis;26,32 in some cases P450 substrates have even been found where the ability of the substrate to induce a type-I response is minimal.34,35 Thus in our binding assay we monitored for three different potential binding modes, with special focus regarding the unusual type-III binding spectra. We then optimised the assay conditions to examine binding of our substrates to OxyBvan, where we determined that the temperature of the assay was very important to observe a spin-state shift (i.e. binding signal) of the P450 (see Fig. S1†); such temperature effects have also been observed in the case of other P450s, including the phenolic coupling P450 CYP121.36 The use of a buffer with a pH of 7–8 was important, as the scattering observed in binding assays performed at lower pH values was dramatically worse.
Binding assays performed using vancomycin hexa- and heptapeptide mimics 5–7 indicated a binding response with the type-III signature, which has been previously observed for hexapeptide-loaded PCP-6 and heptapeptide-loaded PCP-7 substrates with OxyBvan (Fig. 4).26 This type of spectral signature indicates a change in the heme environment of the P450 albeit without displacement of the coordinating water ligand by the substrate to generate a type-I spectrum. Such a spectral signature could be caused by several scenarios: either by replacement of the heme-bound water molecule with one of the phenol groups of the peptide or, and more likely, alteration of the ligand strength of the bound water molecule through coordination with the bound peptide substrate as has been observed in other systems.33,37 The scattering observed during the binding assays was severe, with the more hydrophobic heptapeptide 7 displaying a higher scattering signal than the hexapeptide 5; unsurprisingly, methylation of the hexapeptide (6) led to a further increase in scattering, and as a consequence all further peptide mimics were synthesised without N-terminal methylation.
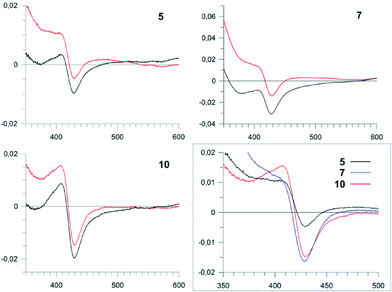 |
| Fig. 4 Difference spectra of the titration of OxyBvan obtained using different peptide linker substrates (5, 7, 10); uncorrected traces and baseline corrected traces are shown in red and black respectively; the box contains a zoomed comparison of the uncorrected traces from 5 (black), 7 (blue) and 10 (red). X-axes: wavelength (nm), Y-axes: absorbance (arbitrary units). | |
Inhibitor design
Given that OxyBvan displays unique and desirable peptide cyclisation properties so far not identified in other Oxy enzymes,12,23,27 it was important to probe how alterations in peptide substrate affect the binding mode observed to OxyBvan. To begin such investigations, we synthesised peptides with histidine substitution of either of the two residues involved in the OxyB-catalysed crosslink formation (residues 4/6). For the substitution of the D-4-hydroxyphenylglycine (Hpg) residue at position four of the peptide we tested the incorporation of both D- and L-histidine (peptides 9 and 10, respectively), as we were using a typical proteogenic amino acid to replace a phenylglycine residue, which possesses an unusual planar side chain.7 In general the behaviour of the histidine-containing hexapeptide mimics was improved over the native peptide sequence, with a reduction in scattering seen for all peptides, which is probably due to the more hydrophilic character of histidine in contrast to Hpg. In terms of interaction with OxyBvan, the substitution of the Hpg residue at position four of the peptide for L-histidine gave the most striking response in binding, displaying the type-III spectrum as discussed above; the KD of the interaction was also comparable to that reported for the PCP substrates (10–20 μM).26 The substitution of the tyrosine residue at position six produced compound 8 that no longer exhibited a strong spectral response from OxyBvan, which led us to conclude that the synthesis of compounds with alternate substituents at position four of the peptide would be the most promising active site probes as well as potential inhibitors. This result also strongly supports the hypothesis that Hpg-4 is the residue directly interacting with the heme group of the P450.
With knowledge of these results, we then synthesised a series of compounds (Fig. 5) bearing different nitrogen containing ligands at position 4 of the peptide (methylated histidine residues (11–12), a pyridyl (13) and a lysine residue (14)). In order to expand the range of possible amino acids available to act as heme-coordinating ligands as well as the “reach” of these compounds, we utilised a selective deprotection procedure to conjugate imidazole carboxylic acid moieties onto linear alkyl amine residues at position 4 of the peptide: thus by synthesising peptides containing diaminopropanoic acid (DAP), ornithine and lysine residues protected by the acid labile 4-methyltrityl (Mtt) group at position 4 we could then selectively deprotect and couple them to imidazole carboxylic acid to produce three additional peptides containing “extended” histidine side chains (15–17, Fig. 6).
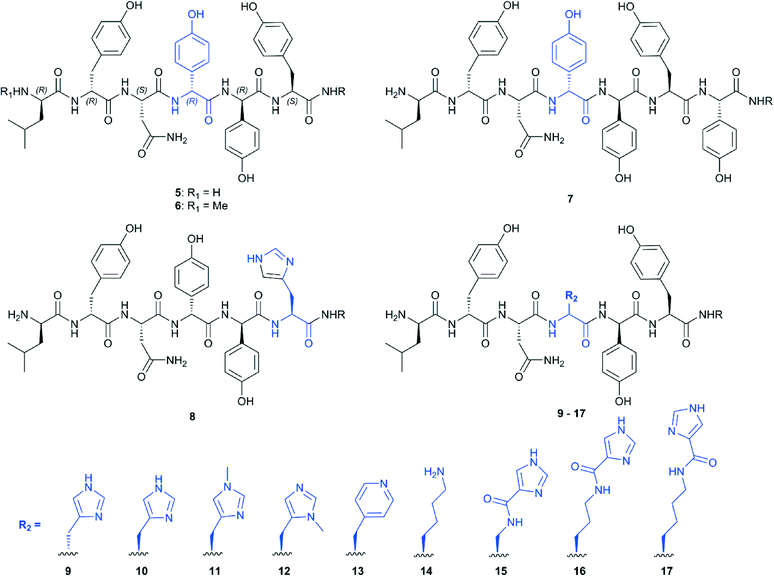 |
| Fig. 5 Linker peptides 5–17 synthesised in this study (R: PPant-linker mimic 1). | |
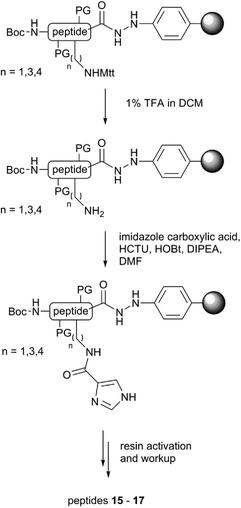 |
| Fig. 6 Synthesis route towards imidazole-conjugated probes 15–17 utilising selective deprotection of precursor amine side chains (PG: Asn – trityl; Tyr – t-butyl). | |
We then examined the binding of these compounds to OxyBvan: the use of a pyridyl residue at position 4 (13) led to the highest scattering observed and did not yield a spectral maximum in the difference spectra (Table 1). Given this difference to the histidine containing peptide, we compared this to N-methylated histidine residues (11, 12) to determine whether the improved binding of the histidine residue could be attributed to a specific nitrogen atom. 11–12 were then tested and showed that 12 triggers a type-I spectral response indicative of the loss of heme coordinated water, most likely due to the steric effects of the methyl group close to the heme iron. 11 affords a much weaker spectral response that is similar to that of the pyridyl compound 13, suggesting that both these compounds do not allow the peptide to bind in an orientation able to trigger either a type-I or type-III response.
Table 1 Binding characteristics of selected probes to OxyBvan
|
Abs (max) |
Abs (min) |
Binding |
Signala (%) |
Scatteringbc |
K
D (μM) |
Signal that is observed from the difference spectra as a percentage of the heme soret absorbance of the P450 prior to titration with the ligands.
Relates the scattering of the titrations at 350 nm – 13 (highest) set as 100%.
Relative scattering: <15% (+), 15–30% (++), 31–50% (+++), 50–100% (++++).
|
5
|
407 |
429 |
Type-III |
5 |
++ |
— |
6
|
— |
— |
— |
— |
+++ |
— |
7
|
405 |
428 |
Type-III |
9 |
+++ |
— |
8
|
— |
430 |
— |
3 |
+ |
— |
9
|
— |
— |
— |
— |
+ |
— |
10
|
407 |
429 |
Type-III |
12 |
+ |
11 ± 2 |
11
|
— |
420 |
— |
6 |
++ |
— |
12
|
387 |
424 |
Type-I |
15 |
+++ |
— |
13
|
— |
429 |
— |
14 |
++++ |
— |
14
|
394 |
429 |
Type-I |
12 |
+++ |
18 ± 1 |
15
|
385 |
427 |
Type-I |
13 |
+ |
16 ± 3 |
16
|
407 |
433 |
Type-III |
14 |
+ |
2 ± 0.5 |
17
|
— |
420 |
— |
4 |
+ |
2 ± 1 |
Investigations of the binding of the peptides containing the larger amino acid residues at position four of the peptide showed differences in behaviour depending upon the length and flexibility of the substituent side chains (Fig. 7): here, the C4-imidazole compound 17 gave a very limited spectral response, whilst the C3-imidazole compound 16 showed a type-III response that is similar to the original imidazole-containing substrate 10. The C1-imidazole compound 15 and lysine-containing compound 14 gave type-I spectral shifts as had been observed for the peptide 12, which indicates that bulky substituents at position four of the peptide generally are able to trigger the loss of the heme bound water molecule in such peptide mimics. Of all these peptide mimics that can trigger a type-I or type-III response, 16 displays the best properties, with a tighter interaction (KD = 2 μM) than is found for the other peptides (10–20 μM) and improved solubility as indicated by low scattering during binding analysis (Table 1).
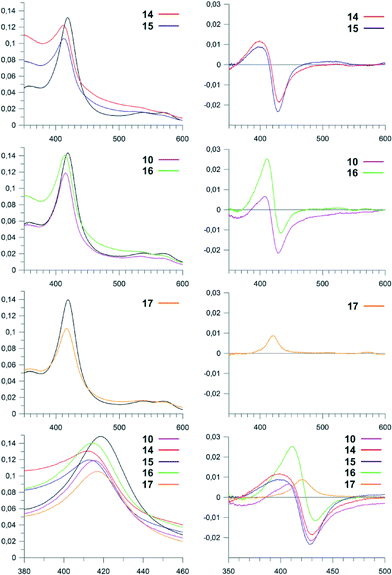 |
| Fig. 7 Absolute (left hand side) and difference spectra (right hand side, baseline corrected traces are shown) obtained upon the titration OxyBvan with most promising peptide linker substrates (10, 14–17) divided into type-I binding mode (top), type-III binding mode (second from top), limited spectral response (third from top) and zoomed comparisons (bottom, reference OxyBvan spectra shown in black). Colours are indicated for each peptide; X-axes: wavelength (nm), Y-axes: absorbance (arbitrary units). | |
Analysis of binding data
The results obtained from the binding studies of the linker peptide compounds discussed here have revealed some interesting properties about the binding of peptide substrates to OxyBvan: firstly, the results support the closest approach of residue four of the peptide to the heme iron and that the type-III spectra is not caused by the direct binding of the phenolic hydroxyl group of the Hpg residue to the heme iron. The closer approach of one aromatic ring is in agreement with structurally characterised examples of P450-substrate complexes from phenolic or aryl crosslinking P450s, where a single aromatic ring of the substrate is positioned close to the heme;36,38,39 computational experiments also suggest that oxidation of the substrates in such orientations rely on proton coupled electron transfer in order to generate a cross-coupling competent diradical state.40–42 Two binding conformations of the aromatic rings have been observed in these structures – one in which the aromatic rings are “stacked”38 and another where the rings are found in a so-called “butterfly” conformation,36,39 although our binding data is not able to suggest which is more relevant to OxyBvan. Curiously, the crystal structure of CYP121 contains both a heme-bound water molecule and substrate molecule and demonstrates the existence of a hydrogen bonding network between them, a situation that may very well be the case for the type-III spectra observed with OxyBvan.36 Secondly, it is clear that unlike in the case of P450s that catalyse the hydroxylation of aminoacyl-PCPs,13,16,25 OxyBvan binding to linker peptides 5–17 can display spectral responses that mimic larger peptide-CP substrates.26,32 The data also shows that the arrangement of the side chain of residue four does not allow direct nitrogen coordination of the heme iron to occur, likely due the side chain adopting a conformation more or less parallel to the heme plane: imidazole and various antifungal P450 inhibitors have been demonstrated to trigger type-II binding responses from OxyB homologues (OxyBtei: ketoconazole (25 μM), miconazole (0.6 μM), clotrimazole 6 (μM), imidazole (1.3 mM)),12,19 so the inability of the linker peptide compounds to elicit this response must be due to their binding mode, rather than an inherent inability of OxyBvan to generate type-II spectra. The binding of these inhibitors is in fact comparable to that of the best linker peptide substrates reported in this study, despite the lack of favourable nitrogen atom coordination to the heme that is present in the binding interaction of standard inhibitors.
The binding data obtained for 5–17 also shows that an increase in bulk of substituent close to the peptide backbone can trigger the loss of the heme bound water, likely due to steric effects. This result helps explains the appearance of either type-I or type-III spectra for OxyBvan binding to different substrates: the natural substrate – a heptapeptide bound to a PCP-X construct – evokes the typical type-I spectra due to the correct positioning of the longer heptapeptide close to the heme iron.23 Synthetic substrate pairings (PCP-6 bound hexapeptides,26 PCP-7 bound heptapeptides26 or hexapeptide-PCP-X di-domain substrates)43 are unable to trigger loss of the heme bound water and thus lead to type-III spectra upon binding: in the case of hexapeptide substrates this is most likely due to the “missing” 7th amino acid residue that then does not allow the peptide to closely approach the heme, whilst in the case of the PCP-7 bound heptapeptides type-III spectra could be caused by the absence of the X-domain to ensure the correct binding of the larger peptide substrate in the P450 active site.
Influence of inhibitor peptides on OxyBvan activity
Based on the binding data obtained, we evaluated the potential of such linker peptides to act as inhibitors of OxyBvan. To investigate this, we performed OxyBvan activity assays in the presence of the most promising linker peptides (10, 14–16) to investigate their effect on C-O-D ring formation of the vancomycin-like hexapeptide 5 bound to the carrier protein from module 7 of complestatin biosynthesis (5-CP) – a PCP domain that in our hands has proven to be a useful vehicle for hexapeptide oxidation by OxyBvan.27 Analysis of the level of C-O-D ring formation observed in these assays showed varying degrees of reduction in OxyBvan activity for all four linker peptides tested (Fig. 8): the addition of the C1-imidazole compound 15 dramatically reduced OxyBvan activity and the lysine-containing peptide 14 completely abolished C-O-D ring formation even after 15 minutes of incubation. Addition of the histidine-containing peptide 10 led to a reduction in C-O-D ring formation by 50%, whilst the C3-imidazole compound 16 had only a minor inhibitory influence on OxyBvan activity. Curiously, the inhibition tendency of these linker peptides does not correlate with the binding affinity; rather it appears linked to binding modes observed for these peptides. Thus, the strongest binding peptide 16 exhibited the lowest inhibitory effect, followed by 10, which both induce a type-III binding response. The more inhibitory potent compounds 14 and 15 induce a type-I response on binding to OxyBvan, which may indicate that these probes are more effective at disrupting the hydrogen bonding network in the active site that in turn leads to improved inhibition of the enzyme. Overall, it is clear that small changes in the substituent on position four of the peptide have a strong influence not only on the binding response but also on the inhibitory potential of these linker peptides. This is especially clear when comparing 10, 15 and 16, which all bear imidazole residues on position four but possess varying linker chain lengths from the imidazole moiety and the peptide backbone. Peptide 14 induced the strongest inhibitory effect on OxyBvan, possible due to the amine moiety of the lysine residue being best able to adopt the position of the phenol group in mimicking the Hpg residue of the natural peptide (C4-spacer between peptide chain and functional group). An alternative suggestion would be that the high flexibility of the lysine alkyl chain present in 14 could allow stabilising interactions of the ε-amino group with other residues in the active site, thus causing the observed inhibition of enzyme activity by this compound. It is clear from these inhibition studies that the structural characterisation of complexes of linker peptides bound to OxyB would greatly assist in understanding the effects noted for the different peptide types and the correlation between the mode of binding observed and the inhibitory effect of these linker peptides.
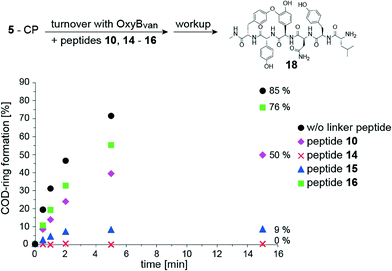 |
| Fig. 8 Investigation of the influence of peptides 10, 14–16, on the in vitro activity of OxyBvan in catalysing C-O-D-ring formation; the vancomycin-derived hexapeptide 5 bound to PCP-7 from complestatin biosynthesis (Trx-PCP7com) was used as a substrate as previously described.27 In the turnover the following reagents were used: 5-PCP (50 μM), OxyBvan (1 μM), peptides 10/14–16 (500 μM), NADH, PuR, PuX, glucose, alcohol-dehydrogenase, air, Hepes buffer pH 7, 37 °C. Workup was performed by adding methylamine after different time points to quench the reaction and release the peptide from the carrier protein; analysis was then performed using LC-MS. Percentage conversion of 5-CP after 15 min is indicated on the right hand side of the graph. | |
Experimental
General methods
All chemicals and solvents were obtained from commercial suppliers (Merck Novabiochem; Sigma-Aldrich; VWR; 1 from Iris-Biotech) and used without further purification. Solid phase peptide synthesis was performed on a Tribute UV synthesiser (Protein Technologies). For solid phase extraction Strata-X SPE columns (Phenomenex) were used. HPLC analyses and purifications were carried out using a LCMS-2020 High Performance Liquid Chromatograph/Mass Spectrometer (ESI,† operating both in positive and negative mode) equipped with a SPD-M20A Prominence Photo Diode Array Detector in preparative mode and a SPD-20A Prominence Dual Wavelength UV Detector in analytical mode (Shimadzu). In analytical mode, LC-20 AD solvent delivery modules were used; for preparative purifications LC-20AP units were used. Analytical analyses were performed on Waters XBridge BEH300 C18 columns (5 μm, 4.6 × 250 mm) at a flow rate of 1 mL min−1. Preparative separations were performed on a Waters XBridge BEH300 Prep C18 column (5 μm, 19 × 150 mm) at a flow rate of 20 mL min−1. Solvents used were water + 0.1% formic acid (solvent A) and HPLC-grade MeCN + 0.1% formic acid (solvent B).
Solid phase peptide synthesis
Solid phase peptide synthesis was performed on 4-Fmoc-hydrazinobenzoyl AM NovaGel resin (0.61 mmol g−1) on 50 μmolar scale employing conditions as previously reported.28
Displacement of the peptide from hydrazinobenzoyl resin using 1
After peptide synthesis, the resin was transferred into a 50 mL falcon tube and swollen in 3 mL of DMF with gentle shaking. 3-Acetamido-N-(2-aminoethyl)propanamide hydrochloride 1 (10 eq., 500 mmol, 104 mg) was suspended in DMF (6 mL), with dissolution upon addition of DIPEA (100–150 μL). This was then added to the resin and Cu(OAc)2 (~5 mg) was added; after this, the reaction was allowed to proceed for 4 h with air bubbled through the mixture. Afterwards, the sample was filtered through celite and the resin thoroughly washed with DMF. After removing the solvent in vacuo, side chain protecting groups were cleaved by incubating the remaining solid in 4 mL of TFA/TIPS/H2O-mixture (95
:
2.5
:
2.5) for 1 h. The solution was concentrated under a stream of nitrogen to ~1 mL and the peptide precipitated via addition of cold Et2O. After centrifugation, the peptide was dissolved in 95% water/5% MeCN and purified using 4 × 500 mg Strata-X SPE cartridges activated with 5 mL of methanol and equilibrated with 3 mL of water in advance. After loading of the peptide sample, the columns were washed sequentially with 3 mL of water, 3 mL of 5% MeOH in water and 3 mL of 5% MeOH in water + 0.1% TFA. Elution of the peptide was performed using 3 mL of 25–30% MeCN in water + 0.1% TFA. The peptide fraction was freeze-dried, further purified using preparative HPLC and analysed using reverse-phase HPLC-MS.
Mtt removal procedure
The resin was swollen in 3 mL of DCM and treated with 3 mL of freshly prepared TFA solution (1% in DCM) for 2 min. The resin was filtered, carefully washed with DCM and immediately treated again with 3 mL of 1% TFA solution for 2 min. This procedure was repeated until no colour change was observed. The resin was washed with DCM (5 × 3 mL) and dried under a stream of nitrogen.
Coupling of 4-imidazolecarboxylic acid
4-Imidazolecarboxylic acid (56 mg, 500 μmol, 10 eq.) was pre-activated with HCTU (207 mg, 10 eq.), HOBt (77 mg, 10 eq.) and DIPEA (65 mg, 85 μL, 10 eq.) in 3 mL of DMF for 5 min. After transferring the mixture to the swollen resin, the reaction was allowed to proceed for 2 h with gentle shaking. After filtering and washing the resin, the coupling step was repeated. The resin was washed thoroughly with DMF and DCM and dried under a stream of nitrogen.
OxyBvan expression and purification
OxyBvan was expressed and purified as previously reported.27
Inhibitor binding assays
UV/visible measurements were performed on a JASCO V-650 double-beam spectrophotometer using a temperature controlled cell. All measurements were performed at 37 °C across an absorbance range of 350–600 nm. 1 ml of enzyme solution (2.5 μM) in 50 mM Hepes buffer (pH 7.0) was divided between a sample and a reference cuvette. Difference spectra were recorded by adding successive aliquots of peptides (5 mM stock solution in water) to the sample cuvette until no further changes were recorded. For each step, difference spectra were recorded after an equilibration time of 3 min. The absolute amplitudes of each spectrum were determined (ΔA = |Amax| + |Amin|) and plotted against the ligand concentration. The resulting data points were fitted to a one-site binding model (Y = Bmax × X/(Kd + X)) to derive the dissociation constant Kd using the program GraFit 7. Baseline correction was performed using a|e – UV-vis-IR Spectral Software 2.2.44
Activity assay with inhibitor peptides
A mixture of peptide 5 loaded onto Trx-PCP7com27 (50 μM), OxyBvan (1 μM), inhibitor peptide (500 μM) together with ferredoxin Pux (5 μM),45 ferredoxin reductase PuR (1 μM),45 glucose (0.33%) and alcohol dehydrogenase (33 μg mL−1) as NADH regeneration system was incubated in Hepes buffer (50 mM Hepes, 50 mM NaCl, pH 7) at 37 °C for three minutes. Turnover was started by the addition of NADH (2 mM) and incubated at 37 °C with gentle shaking. The reactions were stopped at defined time points via the addition of methylamine (40% solution in water). Workup and analysis was performed as described.43
Conclusions
The peptide linker substrates 5–17 synthesised and tested in this study are able to effectively mimic the binding of protein bound peptide substrates to OxyBvan in conformations that evoke either a type-I or type-III spectral response. The substitution of amino acid residues within the peptide provided information about the heme-interacting residue and enabled the design of peptides that act as inhibitors of OxyBvan. The Ppant linker mimic 1 has proven to be a valuable alternative to the more standard SNAc or CoA linkers and offers several advantages over SNAc linkers, such as hydrolytic stability and longer linker length that also results in an increase in polarity. Currently, co-crystallisation studies of OxyBvan with several of the most promising peptide linker probes are underway in an attempt to gain further structural insights into the peptide binding mode and inhibition of this biosynthetically important P450 enzyme.
Acknowledgements
We thank A. Koch (MPI-Hd) for protein expression, K. Haslinger (MPI-Hd) for discussions and K. Keeling (Uni. of Adelaide) for peptide synthesis. M. J. C. is grateful for the support of the Deutsche Forschungsgemeinschaft (Emmy – Noether Program, CR 392/1-1) and the Deutsche Akademischer Austausch Dienst (Group of Eight Australia – Germany Joint Research Co-operation Scheme, grant 56265933).
Notes and references
-
P. R. Ortiz de Montellano, Cytochrome P450 Structure, Mechanism and Biochemistry, Springer, 2015 Search PubMed.
- M. J. Cryle, J. E. Stok and J. J. De Voss, Aust. J. Chem., 2003, 56, 749–762 CrossRef CAS.
-
M. J. Cryle, C. Brieke and K. Haslinger, in Amino Acids, Peptides and Proteins, The Royal Society of Chemistry, 2014, vol. 38, pp. 1–36 Search PubMed.
- M. J. Cryle, Metallomics, 2011, 3, 323–326 RSC.
- M. A. Fischbach and C. T. Walsh, Chem. Rev., 2006, 106, 3468–3496 CrossRef CAS PubMed.
- G. H. Hur, C. R. Vickery and M. D. Burkart, Nat. Prod. Rep., 2012, 29, 1074–1098 RSC.
- R. S. Al Toma, C. Brieke, M. J. Cryle and R. D. Sussmuth, Nat. Prod. Rep., 2015, 32, 1207–1235 RSC.
- G. Yim, M. N. Thaker, K. Koteva and G. Wright, J. Antibiot., 2014, 67, 31–41 CrossRef CAS PubMed.
- A. Kirschning and F. Hahn, Angew. Chem., Int. Ed., 2012, 51, 4012–4022 CrossRef CAS PubMed.
- M. N. Thaker and G. D. Wright, ACS Synth. Biol., 2015, 4, 195–206 CrossRef CAS PubMed.
- L. M. Podust and D. H. Sherman, Nat. Prod. Rep., 2012, 29, 1251–1266 RSC.
- K. Haslinger, E. Maximowitsch, C. Brieke, A. Koch and M. J. Cryle, ChemBioChem, 2014, 15, 2719–2728 CrossRef CAS PubMed.
- K. Haslinger, C. Brieke, S. Uhlmann, L. Sieverling, R. D. Süssmuth and M. J. Cryle, Angew. Chem., Int. Ed., 2014, 53, 1–6 CrossRef PubMed.
- Z. Li, S. G. Rupasinghe, M. A. Schuler and S. K. Nair, Proteins: Struct., Funct., Bioinf., 2011, 79, 1728–1738 CrossRef CAS PubMed.
- M. J. Cryle, J. Staaden and I. Schlichting, Arch. Biochem. Biophys., 2011, 507, 163–173 CrossRef CAS PubMed.
- M. J. Cryle, A. Meinhart and I. Schlichting, J. Biol. Chem., 2010, 285, 24562–24574 CrossRef CAS PubMed.
- M. J. Cryle and I. Schlichting, Proc. Natl. Acad. Sci. U. S. A., 2008, 105, 15696–15701 CrossRef CAS PubMed.
- O. Pylypenko, F. Vitali, K. Zerbe, J. A. Robinson and I. Schlichting, J. Biol. Chem., 2003, 278, 46727–46733 CrossRef CAS PubMed.
- K. Zerbe, O. Pylypenko, F. Vitali, W. Zhang, S. Rouset, M. Heck, J. W. Vrijbloed, D. Bischoff, B. Bister, R. D. Sussmuth, S. Pelzer, W. Wohlleben, J. A. Robinson and I. Schlichting, J. Biol. Chem., 2002, 277, 47476–47485 CrossRef CAS PubMed.
- M. J. Cryle, Biochem. Soc. Trans., 2010, 38, 934–939 CrossRef CAS PubMed.
- J. Crosby and M. P. Crump, Nat. Prod. Rep., 2012, 29, 1111–1137 RSC.
- K. Haslinger, C. Redfield and M. J. Cryle, Proteins: Struct., Funct., Bioinf., 2015, 83, 711–721 CrossRef CAS PubMed.
- K. Haslinger, M. Peschke, C. Brieke, E. Maximowitsch and M. J. Cryle, Nature, 2015, 521, 105–109 CrossRef CAS PubMed.
- S. Pohle, C. Appelt, M. Roux, H.-P. Fiedler and R. D. Süssmuth, J. Am. Chem. Soc., 2011, 133, 6194–6205 CrossRef CAS PubMed.
- S. Uhlmann, R. D. Süssmuth and M. J. Cryle, ACS Chem. Biol., 2013, 8, 2586–2596 CrossRef CAS PubMed.
- K. Woithe, N. Geib, K. Zerbe, D. B. Li, M. Heck, S. Fournier-Rousset, O. Meyer, F. Vitali, N. Matoba, K. Abou-Hadeed and J. A. Robinson, J. Am. Chem. Soc., 2007, 129, 6887–6895 CrossRef CAS PubMed.
- C. Brieke, V. Kratzig, K. Haslinger, A. Winkler and M. J. Cryle, Org. Biomol. Chem., 2015, 13, 2012–2021 CAS.
- C. Brieke and M. J. Cryle, Org. Lett., 2014, 16, 2454–2457 CrossRef CAS PubMed.
- C. R. Millington, R. Quarrell and G. Lowe, Tetrahedron Lett., 1998, 39, 7201–7204 CrossRef CAS.
- J. B. Blanco-Canosa and P. E. Dawson, Angew. Chem., Int. Ed., 2008, 47, 6851–6855 CrossRef CAS PubMed.
- Y.-H. Woo, A. Mitchell and J. Camarero, Int. J. Pept. Res. Ther., 2007, 13, 181–190 CrossRef CAS.
- P. C. Schmartz, K. Wölfel, K. Zerbe, E. Gad, E. S. El Tamany, H. K. Ibrahim, K. Abou-Hadeed and J. A. Robinson, Angew. Chem., Int. Ed., 2012, 51, 11468–11472 CrossRef CAS PubMed.
- N. Mast, W. Zheng, C. D. Stout and I. A. Pikuleva, J. Biol. Chem., 2013, 288, 4613–4624 CrossRef CAS PubMed.
- A. B. Carmichael and L.-L. Wong, Eur. J. Biochem., 2001, 268, 3117–3125 CrossRef CAS.
- C. F. Harford-Cross, A. B. Carmichael, F. K. Allan, P. A. England, D. A. Rouch and L.-L. Wong, Protein Eng., 2000, 13, 121–128 CrossRef CAS PubMed.
- P. Belin, M. H. L. N. Le Du, A. Fielding, O. Lequin, M. L. Jacquet, J.-B. Charbonnier, A. Lecoq, R. Thai, M. Courçon, C. D. Masson, C. Dugave, R. Genet, J.-L. Pernodet and M. Gondry, Proc. Natl. Acad. Sci. U. S. A., 2009, 106, 7426–7431 CrossRef PubMed.
- H. E. Seward, A. Roujeinikova, K. J. McLean, A. W. Munro and D. Leys, J. Biol. Chem., 2006, 281, 39437–39443 CrossRef CAS PubMed.
- B. Zhao, F. P. Guengerich, A. Bellamine, D. C. Lamb, M. Izumikawa, L. Lei, L. M. Podust, M. Sundaramoorthy, J. A. Kalaitzis, L. M. Reddy, S. L. Kelly, B. S. Moore, D. Stec, M. Voehler, J. R. Falck, T. Shimada and M. R. Waterman, J. Biol. Chem., 2005, 280, 11599–11607 CrossRef CAS PubMed.
- M. Makino, H. Sugimoto, Y. Shiro, S. Asamizu, H. Onaka and S. Nagano, Proc. Natl. Acad. Sci. U. S. A., 2007, 104, 11591–11596 CrossRef CAS PubMed.
- V. G. Dumas, L. A. Defelipe, A. A. Petruk, A. G. Turjanski and M. A. Marti, Proteins: Struct., Funct., Bioinf., 2014, 82, 1004–1021 CrossRef CAS PubMed.
- Y. Wang, H. Chen, M. Makino, Y. Shiro, S. Nagano, S. Asamizu, H. Onaka and S. Shaik, J. Am. Chem. Soc., 2009, 131, 6748–6762 CrossRef CAS PubMed.
- Y. Wang, H. Hirao, H. Chen, H. Onaka, S. Nagano and S. Shaik, J. Am.
Chem. Soc., 2008, 130, 7170–7171 CrossRef CAS PubMed.
-
C. Brieke, M. Peschke, K. Haslinger and M. J. Cryle, Unpublished Results, 2015 Search PubMed.
-
S. Preus, a|e - UV-vis-IR Spectral Software 2.2, FluorTools, 2015 Search PubMed.
- S. G. Bell, F. Xu, E. O. D. Johnson, I. M. Forward, M. Bartlam, Z. Rao and L.-L. Wong, J. Biol. Inorg. Chem., 2010, 15, 315–328 CrossRef CAS PubMed.
Footnote |
† Electronic supplementary information (ESI) available: Characterisation of peptide linker probes. See DOI: 10.1039/c5md00332f |
|
This journal is © The Royal Society of Chemistry 2016 |