A human exposome framework for guiding risk management and holistic assessment of recycled water quality
Received
1st February 2016
, Accepted 7th April 2016
First published on 12th April 2016
Abstract
Challenges associated with water scarcity and increasing water demand are leading many cities around the globe to consider water reuse as a step towards water sustainability. Recycled water may be used in a spectrum of applications, from irrigation or industrial use to direct potable reuse, and thus presents a challenge to regulators as not all applications require the same level of treatment. We propose that traditional drinking water standards identifying “safe” water quality are insufficient for recycled water and that using the “human exposome” as a framework to guide development of a risk management strategy offers a holistic means by which to base decisions impacting water quality. A successful and comprehensive plan for water reuse must consider 1) health impacts associated with both acute and chronic exposures, 2) all routes of exposure by which individuals may encounter recycled water, and 3) water quality at the true point of use after storage and transport through pipe networks, rather than at the point of treatment. Based on these principles we explore key chemical differences between recycled and traditional potable water, implications for distribution systems with respect to design and operation, occurrence of chronic contaminants, and the presence of emerging and often underappreciated microbial contaminants. The unique nature of recycled water has the potential to provide rapid regrowth conditions for certain microbial contaminants in these systems, which must be considered to achieve safe water quality at the point of use.
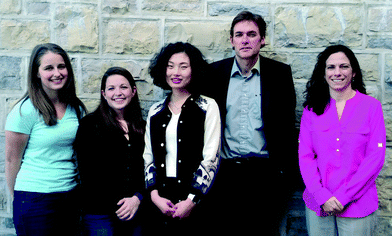
From left to right, the authors in the photograph are Laurel Strom, Emily Garner, Ni Zhu, Marc Edwards, and Amy Pruden
| Emily Garner is currently a Ph.D student at Virginia Tech in the department of Civil and Environmental Engineering. She received a Bachelor's Degree in Civil Engineering from West Virginia University in 2013. Emily is passionate about studying microbiological aspects of water, particularly as they relate to public health. For her graduate work, Emily is studying the presence of emerging microbial contaminants in recycled water systems. She is the recipient of a National Science Foundation Graduate Research Fellowship. She is also a member of the Flint Water Study team from Virginia Tech that worked with citizens of Flint, Michigan to identify unsafe levels of lead in their drinking water. |
| Ni Zhu is a doctoral student in the department of Civil and Environmental Engineering at Virginia Tech. She received her B.S. in Environmental Science and Engineering from National University of Singapore and a Masters of Engineering degree in Civil and Environmental Engineering from Massachusetts Institute of Technology. Her current research area focuses on the effects of water chemistry on the attenuation of emerging microbial contaminants and antibiotic resistant gene/bacteria in recycled water distribution systems, using the next-generation sequencing technology. |
| Laurel Strom is currently is a graduate student at Virginia Tech pursuing a Master's of Science degree in Environmental Engineering. She grew up an hour north of Seattle, WA and received her Bachelor's of Science in Civil Engineering from Washington State University in Pullman, WA in 2014. Her research interests are at the interface of drinking water and microbiology, both through the investigation of drinking water pathogens and in seeking solutions for the biostabilization of drinking water in the United States. |
| Marc Edwards is the Charles P. Lunsford Professor of Civil and Environmental Engineering at Virginia Tech and a MacArthur Foundation Fellow. He is widely considered the foremost authority on lead in drinking water and recently revealed widespread lead contamination in Flint, MI tap water, along with an array of government agency failures that led to the crisis. His current research explores how water chemistry shapes the tap water microbiome and implications for design of water and energy conserving systems in green buildings. |
| Amy Pruden is the W. Thomas Rice Professor of Civil and Environmental Engineering at Virginia Tech. Her research focuses on tracking pathogens and antibiotic resistance genes through environmental systems and developing engineering control strategies for protecting public health. Her broad research mission is to advance the sustainability and health of our water systems through fundamental understanding of microbial ecology. She is the recipient of the Presidential Early Career Award in Science and Engineering and the Paul L. Busch Award for Innovation in water research. |
Water impact
Here, we explore the inherent chemical differences between recycled and potable water and their implications for microbial regrowth in recycled water distribution systems. We propose that the concept of the “human exposome” – the summation of environmental exposures over the course of a lifetime – provides ideal guiding principles for the development of appropriate water reuse risk management strategies.
|
Introduction
Water reuse is essential for satisfying domestic and industrial water demand worldwide and achieving water sustainability.1 Domestic wastewater can be treated to the necessary level of quality and reused to reduce loss of treated effluent via discharge, relieve pressures on depleting groundwater aquifers, and minimize extraction of water from fragile environments such as drought-stricken surface waters. Treatment of wastewater for reuse is also cost effective compared to alternative approaches, such as obtaining freshwater from desalination.2 Particularly when wastewater is treated for direct or indirect potable reuse, a “multi-barrier” treatment framework is typically used to ensure that multiple means of removing pathogens or harmful chemicals will protect public health in the case of a process failure or other unexpected event that could compromise water quality.3 However, while this approach is logical for controlling acute health threats associated with water as it leaves the treatment facility, it does not address concerns with respect to low-level chronic exposures or changes in water quality during distribution to the point of use.4
A major concern for water reuse in general is the lack of federal regulations.5 Further, the few nascent recycled water quality regulations and guidelines available, typically at the state and local level, have been narrowly focused on fecal indicator bacteria (i.e., total and fecal coliforms).6–8 This approach addresses traditional concerns regarding fecal-associated pathogens, but does not necessarily provide insight into safeguarding microbial water quality during distribution.9,10 In particular, microbial contaminants that are of concern due to their ability to grow within distribution systems, such as opportunistic pathogens (OP), antibiotic resistant bacteria and their associated antibiotic resistance genes (ARG), and free-living amoebae (FLA), have little or no relationship with fecal indicator bacteria based standards. Alternative frameworks for assessing and managing recycled water quality more holistically are emerging. For example, adaptations of the hazard analysis and critical control point (HACCP)11 paradigm, which originated in the food safety industry, and the World Health Organization's (WHO) water safety plan (WSP)2,12 have been proposed for use as risk management frameworks for recycled water. Application of these adaptable frameworks could have the advantage of drawing attention to the entire treatment process, rather than focusing solely on absence of indicator organisms as a proxy for safe water. Still, we identify three key elements that should be taken into account for a truly comprehensive consideration of public health concerns: 1) evaluation of health impacts associated with both acute and chronic exposures; 2) accounting for all routes of exposure by which individuals may encounter contaminants in recycled water; and 3) consideration of water quality at the true point of use after storage and transport through pipe networks, rather than at the point of treatment.
A more holistic approach to characterizing the physical, chemical, and microbial characteristics of recycled water, as well as the routes by which humans are exposed, can be derived from the emerging concept of the “human exposome.” The exposome has been defined as “the cumulative measure of environmental influences and associated biological responses throughout the lifespan, including exposures from the environment, diet, behavior, and endogenous processes.”13 The exposome includes general (e.g., climate, urban environment), specific (e.g., water, food, air), and internal (e.g., metabolism, gut/lung microbes) factors and their role in disease.14 Clearly, water, and its corresponding chemical and microbial properties is a fundamental component of the exposome. Water is fundamental to human health, survival, and hygiene and is an integral part of daily life, including direct contact (i.e., drinking, cooking, and showering) and indirect exposures via bioaerosols (i.e., cooling water, flush toilets, or lawn irrigation). We propose that adopting the exposome paradigm as a model for recycled water quality assessment can be used to guide development of an HACCP, WSP, or other comprehensive risk management strategy that more accurately reflects the true risks and exposures associated with water reuse and can strengthen implementation of existing risk management strategies.
In terms of safeguarding recycled water from the point of treatment to the point of use, we note that there are important distinctions between potable and recycled water that should not be ignored, particularly with respect to design, operation, and maintenance of recycled water distribution systems (RWDS). In this critical review, we note key chemical differences between recycled and potable waters, with a particular emphasis on organic matter, and explore implications for RWDSs with respect to design, operation, and intended application. We also discuss how these key differences impact the presence of chronic contaminants and emerging concerns about the presence of OPs, ARGs, and other microbial contaminants (Fig. 1). Our goal is to proactively address plausible public health risks associated with practical realities of recycled water use.
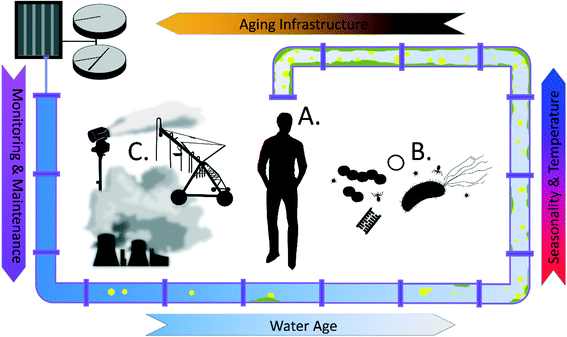 |
| Fig. 1 Key aspects of the exposome paradigm for managing RWDS emphasize holistic consideration of potential exposures to recycled water, including A) chemical distinctions of recycled vs. traditional potable water, such as enriched organic matter/nutrients, disinfectant decay, critical reactive zones and chronic contaminants; B) emerging concerns about ARGs, OPs and other microbial contaminants; C) nontraditional routes of exposure, including inhalation, dermal contact. | |
Unique aspects of RWDS design, operation, and water use
There is a broad continuum of applications for water reuse, ranging from unintended de facto reuse to direct potable reuse (DPR) produced by advanced treatment processes. De facto reuse refers to a situation where reuse of treated wastewater occurs but is not planned, for example, when a drinking water treatment plant intake is located downstream from a wastewater discharge.6 In the U.S., de facto reuse is widespread and becoming increasingly common in recent decades. Rice et al. found that of the top 25 drinking water treatment plants most impacted by upstream wastewater treatment plant (WWTP) discharges in the U.S., the fraction of their water source comprised of WWTP discharges increased from between 2 to 16% in 1980 to an average of 68% under typical streamflow conditions in 2008.15 Some treatment plants received as much as 100% WWTP effluent under low flow conditions. Indirect potable reuse refers to the use of treated wastewater to augment other potable source waters following retention in an environmental buffer.6 Common environmental buffers include groundwater aquifer recharge and subsequent withdrawal prior to drinking water treatment or intentional discharge of wastewater effluent upstream or into a reservoir from which water is withdrawn for drinking water treatment. DPR consists of treating wastewater for direct use as a source water for drinking water treatment. DPR is currently limited in full scale application, with Windhoek, Namibia16 and Big Spring, Texas, USA17 serving as prime examples, but there is growing interest in expanding DPR infrastructure in the U.S.
Wastewater may also be treated for non-potable reuse when it can offset water demand associated with landscape or recreation area irrigation, agricultural and food crop irrigation, snowmaking, industrial use such as in cooling towers or in natural gas production, or to augment environmental waters such as in groundwater aquifer recharge, river or stream flow augmentation, or in wetlands.6 Though treatment requirements may reasonably be lower in these cases, all of these non-potable reuse scenarios have relevant human exposures that should be taken into consideration.
Table 1 illustrates that there is a wide range of observed recycled water quality characteristics as a function of increasing levels of treatment. Unlike drinking water, where consistent regulations are applied, recycled water is used for a wide spectrum of applications with required treatment varying based on intended use. For example, the most stringent requirements are applied to DPR, whereas residual nutrients can be viewed as a beneficial fertilizer in non-potable reuse scenarios. Hence, efficient reuse treatments ideally match the intended purpose of the recycled water. A number of U.S. states pioneering water reuse have recognized this concept of “fit-for-purpose,” tailoring recycled water treatment regulatory guidelines based on end uses. Determining the ideal configuration of treatment processes for different reuse scenarios would greatly benefit from research integrating water treatment outcomes with the exposome paradigm for more comprehensively considering chemical and microbial risks.
Table 1 Water quality as a function of treatment processes
Water quality parameter (units) |
Treatment processes |
Conventional activated sludge (CAS) effluent |
CAS with filtration |
CAS with biological nutrient removal |
CAS with biological nutrient removal and filtration |
Membrane bioreactor |
Filtration with chlorine |
CAS with filtration and chlorine |
Membrane bioreactor with chlorine |
Membrane bioreactor with UV/ozone |
Source ref. 29 and 192.
|
Turbidity (NTU) |
2–15 |
0.5–4 |
2–8 |
0.3–2 |
≤1 |
1.5–8.7 |
3.6–6.3 |
1.7–4.3 |
0.2–0.5 |
Total suspended solids (mg L−1) |
5–25 |
2–8 |
5–20 |
1–4 |
<2 |
|
|
|
|
TOC (mg-C L−1) |
10–40 |
8–30 |
8–20 |
1–5 |
0.5–5 |
12–16 |
6–8 |
3–5 |
2–3 |
BDOC (mg-C L−1) |
|
|
|
|
|
5–7 |
1–2 |
1–2 |
<1 |
AOC (mg-C L−1) |
|
|
|
|
0.2–1.4 |
1–2 |
1–2 |
1–2 |
<1 |
Total nitrogen (mg-N L−1) |
15–35 |
15–35 |
3–8 |
2–5 |
<10 |
5–10 |
<1 |
1–3 |
5–6 |
Total phosphorus (mg-P L−1) |
4–10 |
4–8 |
1–2 |
≤2 |
<0.3–5 |
3–5 |
1–2 |
5–9 |
<1 |
Volatile organic compounds (μg L−1) |
10–40 |
10–40 |
10–20 |
10–20 |
10–20 |
|
|
|
|
Total coliforms (CFU per 100 mL) |
104–105 |
103–105 |
104–105 |
104–105 |
<100 |
<1 |
1–10 |
1–10 |
1–10 |
Protozoa and cysts (CFU per 100 mL) |
10–102 |
0–10 |
0–10 |
0–1 |
0–1 |
1–10 |
10–100 |
<1 |
≤1 |
Viruses (CFU per 100 mL) |
10–103 |
10–103 |
101–103 |
10–103 |
1–103 |
Present |
Present |
Present |
Negative |
Routes of exposure
Stemming from the continuum of recycled water uses described above, there is also a range of relevant exposure scenarios (Table 2). Use of a traditional water quality paradigm based on monitoring of fecal indicator bacteria as a sole benchmark for establishing safe water quality neglects risk associated with non-fecal pathogens and routes of exposure other than ingestion. Though ingestion and aspiration are potential modes of exposure associated with DPR, inhalation and dermal contact are important, yet often overlooked, exposure routes for potable water and even more so for recycled water. Recycled water is commonly used for purposes that generate inhalable aerosols, including use in cooling towers,18 spray irrigation,19,20 fire-fighting,21 toilet flushing,22 as well as for aesthetic purposes, such as in decorative fountains.23–25 Importantly, relevant exposure zones may be vast, with Legionnaire's disease infection associated with inhalation of aerosols from cooling towers located more than a mile away.18 There is also a strong likelihood of dermal contact when recycled water is used for irrigation in public recreation areas. This may be particularly relevant in irrigated parks, athletic fields, and snowmaking for recreational purposes. Dermal abrasions or other lacerations that may be pre-existing or occur during use of these facilities create an additional route of exposure for infection. When recycled water is used for food crop irrigation, chemical and microbial constituents may also be transmitted to humans on or within crops via ingestion.26 When recycled water is used for DPR, a number of often overlooked routes of exposure should also be considered, for example, including use in humidifiers, ice machines, and decorative water features. A more holistic characterization of human exposures to recycled water constituents via such non-conventional routes is important for accurate assessment of health risk associated with use of recycled water.
Table 2 Overview of non-traditional routes of exposure for recycled water and putative risk of infection or exposure
Route of exposure |
Recycled water application (in addition to other potable uses) |
Putative hazard |
Infectious dose |
Documented concentrations in recycled water |
Documented concentrations in drinking water |
[Percent samples positive (positive concentration range)] |
Oral route of infection.
Based on Pseudomonas spp.
Based on Acanthamoeba keratitis.
MRSA = methicillin resistant Staphylococcus aureus.
Putative hazards consider both Legionella pneumophila and other pathogenic Legionella.
Based on detection of Legionella spp.
Based on Mycobacterium avium.
TTHM = total trihalomethanes.
|
Dermal contact |
Snowmaking, irrigation of athletic and recreation facilities, toilet flushing22 |
Opportunistic pathogen infection of wounds
|
Staphylococcus aureus
107
|
|
17%193 |
6.25%194 |
Pseudomonas aeruginosa
108
|
108–109 colony forming units (CFU)a,195 |
88% (1 ± 1–9 ± 10 CFU per 100 mL)b,29 |
5.6% (up to 700.3 ± 158.7 gene copies per mL)111 |
Acanthamoeba spp.169 |
104 trophozoitesc,196 |
|
Not detected111 |
Cyanobacteria toxicity181 |
|
(up to ∼140 μg mL−1 chlorophyll)197 |
|
Antibiotic resistant infections198 |
|
8% for MRSA of 17% for susceptible S. aureusd,193 |
|
Inhalation and aspiration |
Cooling towers, spray irrigation, toilet flushing,22 fire suppression,21 car washing199 |
Opportunistic pathogen infection of lungs
|
Legionella pneumophila
,
20
|
103–106 CFU200 |
81% (0.4 × 103 ± 0.2 × 103–3.5 × 103 ± 16 × 103 CFU per 100 mL)f,29 |
5.6% (up to 219.4 ± 23.8 gene copies per mL)111 |
Mycobacterium spp.201 |
104–107 CFUa,g,195 |
|
98.1% (2.1 ± 104–4.2 ± 103 gene copies per mL)111 |
Pseudomonas aeruginosa
202
|
108–109 CFUa,195 |
88% (1 ± 1–9 ± 10 CFU per 100 mL)b,29 |
5.6% (up to 700.3 ± 158.7 gene copies per mL)111 |
Staphylococcus aureus
19
|
|
17%193 |
6.25%194 |
Naegleria fowleri
168,169
|
103–105 trophozoites168,203 |
|
8–27%168 |
Cyanobacteria toxicity181 |
|
(up to ∼140 μg mL−1 chlorophyll)197 |
|
Antibiotic resistant infections19 |
|
8% for MRSA of 17% for susceptible S. aureusd,193 |
|
Fecal pathogens |
|
|
|
Disinfection byproducts79 |
|
(9.70–399.37h μg TTHM L−1 79) |
|
Nasal aspiration |
Recreation, |
Opportunistic pathogen infection
|
Potable reuse |
(Sinus irrigation) |
Naegleria fowleri
168
|
|
|
8–27%168 |
Eye and ear contact |
Recreation, direct potable reuse, indirect potable reuse |
Opportunistic pathogen infection
|
Acanthamoeba keratitis
169
|
104 trophozoi-tes196 |
|
|
Pseudomonas aeruginosa
108
|
108–109 CFUa,195 |
88% (1 ± 1–9 ± 10 CFU per 100 mL)b,29 |
5.6% (up to 700.3 ± 158.7 gene copies per mL)111 |
Colonization and delayed infection |
Various |
Antibiotic resistant infections119–121 |
|
8% for MRSA of 17% for susceptible S. aureusd,193 |
|
Opportunistic pathogen infection
|
Pseudomonas aeruginosa
108
|
108–109 CFUa,195 |
88% (1 ± 1–9 ± 10 CFU per 100 mL)b,29 |
|
Staphylococcus aureus
107
|
|
17%193 |
6.25%194 |
Physical and operational issues
Water and wastewater infrastructure degradation has become one of the leading threats to public health and water security.27 Aging and poorly managed pipes can lead to a drastic decrease in water quality of the transported water, arising from complex interactions among chemical, physical, and microbial constituents. New design considerations are needed to ensure sustainable management of recycled water infrastructure, considerate of their distinct physical and operational characteristics relative to potable water systems.
In a recent survey of 71 recycled water systems in the US and Australia, Jjemba et al. identified infrastructure issues as the most prevalent problem associated with managing and maintaining water quality in RWDSs. Over 20% of recycled water facilities listed infrastructure integrity as a water quality concern. The extensive list of infrastructure challenges revealed by the survey includes infrastructure deterioration from high chlorine residual, maintenance of desired pressure and flow during low and inconsistent usage, lack of redundant design and storage, complicated branched distribution systems designed to supply multiple recycled applications from a centralized treatment plant, high corrosivity of water damaging metal pipes, and effective monitoring of the chemical and microbial quality.4 For example, to control microbial activity resulting from nutrient-rich recycled water, up to 40 mg L−1 chlorine has been used in some systems, which can potentially result in widespread damage to water infrastructure.28 Use of reservoirs as a way to satisfy on-demand recycled water applications has also been observed to be a challenge, with impaired water quality resulting from long stagnation time and proliferation of algae and aquatic vegetation.29 Experience from potable water systems has also shown that interaction of iron pipes with water containing high organic content and oxygen tends to promote iron release, producing unacceptable discolored water following stagnation.30 It is important to bear in mind that a shift in water chemistry can have disastrous unintended consequences for corrosion,31–33 and given the unique chemistry of recycled water (Table 3), it will be especially critical to bear this in mind. For example, previous studies have documented cases where switching potable water pipes to DPR pipes resulted in destabilization of the of existing corrosion scales and biofilms and an undesirable degradation of water quality at the point of use.34 Despite the unresolved challenges associated with transporting recycled water, this alternative type of water also presents a creative opportunity for solving the challenging issue of leaking pipes. Tang et al. have successfully demonstrated the autogenous repair phenomenon in copper and iron pipes in drinking water distribution systems (DWDS) via beneficial corrosion deposition.35 Optimistically, with a more diverse water chemistry profile, recycled water may be an even better candidate for protecting aging pipes.
Table 3 Comparing water quality of typical drinking water vs. different recycled water applications
Parameter |
Drinking water |
Recycled water applications |
Implications for distribution |
Private, urban and irrigation |
Direct environ-mental reuse |
Indirect potable recharge |
Industrial applica-tions |
Source: ref. 6, 208 and 209.
WHO guidelines for drinking water quality.
Based on 7 day median with none >800 per 100 mL.
Based on 7 day median with non >14 per 100 mL.
|
Carbon source |
Total dissolved solids (mg L−1) |
500 |
|
-Provides the most limiting nutrient source for bacterial regrowth in distribution systems; |
Chemical oxygen demand (mg L−1) |
|
100 |
70–100 |
70–100 |
70 |
-Consumption of carbons in the distribution system is observed to relate with increased bacterial activity29,204 |
Biochemical oxygen demand (mg L−1) |
|
10–20 |
10–20 |
|
10 |
|
pH |
6.5–8.5 |
6–9 |
6–9 |
7–9 |
7–8.5 |
|
DO (mg L−1) |
Near saturation |
>0.5 |
>3 |
>8 |
>3 |
|
Total suspended solids (mg L−1) |
N/A |
10 |
10 |
|
10 |
|
Chlorine residual (mg L−1) |
<4 |
0.2–1.0 |
0.05 |
|
0.05 |
-Control bacterial growth in the distribution system; |
-Excess chlorine can cause carbon fragmentation and DBPs formation |
-Chlorine may exacerbate antibiotic resistance147,148 |
Total Kjeldahl N (mg L−1) |
<10 |
15–20 |
10–20 |
|
10 |
-Concerns for nitrification and denitrification |
Ammonia-N (mg L−1) |
< 0.2a |
2–20 |
1.5 |
0.2 |
1.5 |
-Concern for nitrification |
Total phosphorus (mg L−1) |
|
2–5 |
0.2 |
|
0.2 |
-Eutrophication and degradation of water quality |
Iron (mg L−1) |
0.3 |
2 |
2 |
|
|
-Caused “red water” |
-Promote growth of corrosion bacteria and damage pipe integrity |
-May select for antibiotic resistant bacteria205 |
Copper (mg L−1) |
1.0 |
0.2–1.0 |
0.2–1.0 |
|
|
-Promote growth of certain corrosion bacteria |
-Toxic to certain bacterial and aquatic species at elevated levels |
-May select for antibiotic resistant bacteria143,206 |
Zinc (mg L−1) |
5 |
0.5–2 |
0.5–2 |
|
|
-May select for antibiotic resistant bacteria143,206 |
Pesticide (mg L−1) |
|
0.05 |
0.05 |
|
|
-May select for antibiotic resistant bacteria207 |
Fecal coliforms (CFU per 100 mL) |
Zero |
Zero |
<200b |
Zeroc |
<200a |
Indicator bacteria for pathogenicity of water |
Temperature, as an overarching parameter, is another critical factor that could have profound implications in designing and monitoring water reuse systems.36 Not only is temperature directly related to microbial activity, disinfectant residual decay, corrosion rate, and dissolved oxygen levels, it is also indirectly linked to consumption patterns, flow patterns and velocity, and bulk water and biofilm interactions. Elevated recycled water temperatures may stem from extended stagnation times, particularly during the day in cases where irrigation is conducted at night to limit evaporation, as well as from use of above-ground pipelines, which facilitate transport of recycled water over long distances.
For on-demand non-potable water reuse applications, such as agricultural irrigation, landscaping, and toilet flushing, many studies have observed distinct consumption variations in daily and seasonal demand patterns.37,38 For example, on a daily scale, the generation of wastewater effluent usually peaks in the daytime when people are active, but the demand of irrigation water usually occurs at night with an offset time of approximately 12 hours. Discrepancies in user patterns makes water stagnation and storage, along with associated water quality deterioration, a prominent concern in design and maintenance of recycled systems. Multiple studies have also documented water quality deterioration during winter or high rainfall periods in systems largely used for irrigation, due to low user frequency.39
True water age may differ substantially from the designed hydraulic residence time of the recycled water systems based on the actual end-use applications. Emerging work in premise (i.e., building) plumbing systems, i.e. the water pipe networks within homes and buildings, has highlighted unique systematic features in terms of longer stagnation time, elevated temperature, and loss of disinfectant residual, which serve to stimulate microbial proliferation precisely at the point of use, thus amplifying any potential exposure risk to end users.40,41 Similar investigations are needed to quantify the risk of exposure associated with user-driven demand patterns in non-potable reuse systems.
Important chemical differences anticipated between recycled and potable water distribution systems
Organic matter
One of the most distinctive characteristics of recycled water is the nature of dissolved organic matter (DOM) and its occurrence at elevated levels. The organic matter in typical potable waters consists of natural organic matter (NOM) derived mostly from oil, planktonic and vegetative matter, and decay by-products in natural water sources. However, it is important to note that DOM present in recycled waters may be quite distinct from that of potable water due to different sources and treatment processes. In a recent review comparing organic matter data published in the last 15 years for drinking water and recycled water systems, Hu et al. identified four distinct classes of NOM in recycled water: recalcitrant DOM, soluble microbial products from biological wastewater treatment units, transformation products from advanced treatment, and emerging contaminants associated with anthropogenic activities.42 It was concluded that DOM composition differed significantly between recycled water and drinking water evaluated against five critical chemical indicators: dissolved organic carbon (DOC), dissolved organic nitrogen, assimilable organic carbon (AOC), estrogenic activity, and disinfection byproduct (DBP) formation potential (Fig. 2). DOC in drinking water ranged from 1.5–11.2 mg L−1 with a median of 3.9 mg L−1 while that in recycled water ranged from 3.6–14.6 mg L−1 with a median of 7.5 mg L−1, indicating recycled water as a much more nutrient rich environment for microbial regrowth and byproduct formation. The heightened levels of biotoxicity, in terms of estrogenic levels, is also widely reported in studies examining effluent organic matter compositions, suggesting a potential health risk when used for recycling applications.43
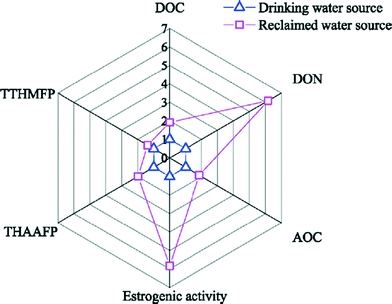 |
| Fig. 2 Overview of typical normalized composition and potential magnitude of dissolved organic matter (DOM) in drinking water sources compared to recycled water sources, presented in terms of dissolved organic carbon (DOC), dissolved organic nitrogen (DON), assimilable organic carbon (AOC), estrogenic activity, total haloacetic acid formation potential (THAAFP), and total trihalomethane formation potential (TTHMFP). (Reprinted from Sci. Total Environ., 551–552, Hong-Ying Hu, Ye Du, Qian-Yuan Wu, Xin Zhao, Xin Tang, Zhuo Chen, differences in dissolved organic matter between reclaimed water source and drinking water source, page 133–142, 2016, with permission from Elsevier.) | |
Biological stability, i.e., the ability of drinking water to suppress microbial growth in the absence of disinfectants,44 is especially of concern for safe transport and storage of treated water. Ideally, low nutrient water will limit growth in the distribution system, a strategy applied successfully in some European countries for eliminating the need for secondary disinfectant in DWDSs.45 The proportion of DOC that facilitates bacterial regrowth is typically measured by either biodegradable dissolved organic carbon (BDOC) or AOC assays. An array of methods have been used to best evaluate the bacterial growth potential of various types of water samples, with established approaches generally being to measure the decrease in measured DOC over time or an increase in indicator bacteria counts as a proxy for biologically available DOC. To date, there is no widely accepted standardized method to quantify biostability. Reported threshold BDOC and AOC values to achieve biostability in drinking water systems using different methods are documented in Table 4. Existing surveys of recycled water systems have indicated orders of magnitude higher levels of organic carbon than in typical U.S. drinking water systems.46,47 In particular, biodegradable organic matter has been observed to be four or five times higher than that of drinking water,29 while AOC can range from 505 to 918 μg L−1 in moderately treated recycled water,48 compared to 18 to 189 μg L−1 in drinking water.49
Table 4 Proposed threshold values to achieve biostability in drinking water distribution systems
Carbon source |
Threshold values |
Criteria |
Ref. |
BDOC |
≤ 0.15 mg-C L−1 |
Stable BDOC values |
210–212
|
≤ 0.25 mg-C L−1 |
213
|
≤ 0.30 mg-C L−1 at 15 °C |
210
|
≤ 0.15 mg-C L−1 |
No coliform growth |
214
|
AOC |
10 μg-C L−1 |
No heterotrophic plate count growth |
215
|
50 μg-C L−1 |
No coliform growth |
216
|
50 μg-C L−1 |
No V. cholerae growth |
217
|
100 μg-C L−1 |
No E. Coli growth |
49
|
The abundance and type of biodegradable carbon in recycled water calls into question the extent to which the science of potable water delivery is directly translatable to recycled water distribution. In the only available study of its kind, Jjemba et al. examined four RWDSs in the U.S. and observed a trend of AOC and BDOC consumption with increasing residence time, with an average reduction of 475 μg L−1 AOC and 370 μg L−1 BDOC from the distribution system point of entry to the point of use.29 They concluded that the change in AOC and BDOC was due to enhanced microbial activity, indicating significant changes in both the quantity and the quality of the available carbon in the RWDSs. In parallel simulated RWDS loop studies, high organic carbon was also observed to result in rapid consumption of disinfectant residuals in the distribution systems.29 Up to 6 mg L−1 of chlorine was completely consumed within minutes in all systems, leaving the remainder of the distribution system vulnerable to bacterial growth.29
Given the unique nature of organic matter and microbial composition of recycled water, existing assays such as those for AOC or BDOC, may not be suitable. Only one study could be found specifically aimed at adapting the AOC assay to recycled water.50 By including test strains that are more ecologically representative of the sample waters, Zhao et al. concluded that the standard P17 and NOX strains applied in the AOC assay largely underestimate levels in recycled water.50 Khan et al. have similarly highlighted the need to optimize the BDOC method for recycled water with their modified protocol improving repeatability and precision of results as verified by independent biochemical oxygen demand and chemical oxygen demand measurements.51
Another negative consequence of NOM in distributed water is that it can accelerate biocorrosion of pipes, which in turn can further stimulate AOC generation.39,52,53 BDOC is also believed to play an important role in microbiologically induced corrosion.54,55 Recycled water, as an abundant source of sulfate and nitrogen species, is likely to provide a nutrient-rich environment for iron-oxidizing/reducing bacteria28,56,57 and sulfate-reducing bacteria34 to thrive in the DS, further raising concerns about the potential for recycled water to accelerate damage to pipe networks.
Redox zones and degradation of water quality
The distribution system can be thought of as a complex reactor with interrelated chemical and biological reactions occurring spatially and temporally as the water passes through the pipes.58 The chemistry of treated potable water changes significantly during transport, with deteriorating DWDS water quality documented since the early 1920s.59–64 Masters et al. illustrated the water distribution system reaction phenomenon by demonstrating the formation of sequential redox zones as a function of water age in simulated DWDS.65 Given greater physiochemical and microbial complexity in RWDS, we speculate that they would foster development of even more dramatic reactive zones, as a function of key physical and hydraulic design parameters such as residence time, flow pattern, hydraulic surface to volume ratio, and pipe layout. Consistent with this expectation, studies in lab and field-scale RWDS recently demonstrated elevated microbial activity as indicated by rapid AOC/BDOC consumption, even at the earliest water age, and attenuated organic carbon at higher water ages.29 Similarly in a 15 month monitoring study of RWDSs, the general pattern observed was an initial reactive zone where rapid microbial regrowth and chemical reactions occurred followed by relatively constant microbial and chemical reactivity along the length of the pipes.66 Recognizing the reality of reactive zones in distribution systems and more deliberately monitoring them may provide valuable insight into predicting and preempting potential problems resulting from issues related to water chemistry.
Disinfectant residual
The intricate relationship between chlorine-based disinfectants and microbial and chemical stability has been intensely studied in DWDSs. Due to its strong oxidizing power, chlorination is generally the disinfectant of choice for microbial control in drinking water treatment. Chlorination can greatly reduce general bacterial counts and help satisfy drinking water microbial regulations. However, as a strong oxidizing agent, it is also known to interact with reductive species, metals, organic matter and pipe materials and, as a result, significantly impact the downstream water chemistry.67 The most widely noted issue with disinfectants is the fragmentation of complex carbon compounds, thus increasing the fraction of biologically available carbon when high concentrations of chlorine are used.29,46,47 Given the tendency to use fecal indicators as a benchmark for assessing recycled water quality, it can be tempting for utilities to dose high concentrations of chlorine. Due to a higher chlorine demand typical of recycled water, disinfectant residual may be rapidly lost, leaving the rest of the RWDS vulnerable to microbial instability.66 Also important to note is that there is growing concern regarding the efficacy of chlorine-based disinfectants against emerging resistant pathogens, which might be more abundant in recycled water than traditional potable water.68–71 The potential for indiscriminate use of disinfectants to inadvertently select disinfectant-resistant bacteria in the RWDS is worthy of exploring in future research. Further, the ability of bacteria to repair and recover in the distribution system following the shock of ultraviolet irradiation (UV), chlorine, or other disinfectant should be considered, as exemplified by recovery of viable but non-culturable bacteria.72–74
Another issue worthy of consideration is the potential for enhanced DBP formation in recycled waters.75,76 In a study comparing DBP formation between wastewater effluent and surface water, Sirivedhin and Gray found that effluent-derived organic matter stimulated formation of higher proportions of brominated DBPs.77 Nurizzo et al. evaluated the DBP formation potential with various disinfection agents and concluded that hypochlorite yielded the greatest total trihalomethanes, exceeding the Italian regulation for agricultural reuse, even when starting with high quality recycled water.78 While DBPs tend to be ignored in recycled waters, particularly for non-potable applications, it is important to recognize that inhalation is also a relevant exposure route to consider, with one model characterizing the inhalation exposure to trihalomethanes of irrigation workers using recycled water suggesting that there was a 13% risk of exceeding acceptable exposure levels for cancer risk.79 The DBP issue illustrates that there can be tradeoffs between microbial control and chemical risks and that clearer guidance and alternative approaches are needed for recycled water to avoid negative consequences of blindly over-chlorinating.
Chronic contaminants
The exposome highlights the importance of considering exposures over the course of one's lifetime, and thus, chronic contaminants are an important hazard worthy of consideration during risk assessment of recycled water. WWTPs are generally not designed with the intention of removing micro-constituents, such as pharmaceuticals and personal care products, recalcitrant organic compounds, heavy metals, nanomaterials, and industrial agricultural additives.80–84 Jelic et al. was able to detect 29 pharmaceutical products in the final effluent of one WWTP, versus 32 in the influent.83 Even when discharged at micro-concentrations, up to hundreds of nanograms per liter of targeted micropollutants can still be consistently detected in receiving water bodies and levels can accumulate.80 In a study that monitored 15 different WWTPs generating recycled water for groundwater recharge, detectable levels of all 20 most commonly used antibiotics were still found at elevated concentrations of 212–4035 ng L−1 in recycled water and 19–1,270 ng L−1 in groundwater.85 Several studies have also observed seasonal patterns of higher discharge of pharmaceuticals and personal care products in wastewater effluent during low flow and less during high flow periods.86,87
While increasing research attention and regulatory efforts have been devoted to understanding prevalence of non-conventional chemical constituents in WWTPs and in receiving environments,88 studies specifically focusing on characterization and risk assessment of emerging chemical constituents of concern in the context of recycled water applications are limited.89–91 Advanced oxidation processes are particularly promising for removal of these pharmaceuticals and other organic compounds (Table 5).92,93 Negative ecological effects of chemical constituents on the aquatic environment have received much attention.94,95 Although a multi-barrier approach consisting of sequential treatment processes has promise, questions remain regarding the ideal treatment for various contaminant types and reasonable end point concentrations that are protective of human and ecological health. Given the diverse applications of recycled water, relevant, accurate and comprehensive risk models are needed considerate of the various environmental spheres of influence. Wastewater effluent discharged to surface water has resulted in detection of emerging pharmaceutical products in 80% of surface water samples.96 Thus, the science and practice of distributing recycled water should proceed with a comprehensive approach to understanding of the fate and impacts of these emerging contaminants in relevant environments.
Table 5 Case studies of existing application of advanced treatment processes for intended reuse purposes. Treatment trains rely on use of biological activated carbon (BAC), reverse osmosis (RO), ultrafiltration, and UV
Advanced treatment processes |
Intended reuse |
Key results |
Ref. |
Ozone/H2O2 + BAC |
Piloted indirect potable reuse |
• H2O2/ozone process demonstrated higher than 90% average removal rate in 21 of 31 targeted trace organic contaminants and hormonal products |
218
|
• BAC unit achieved higher than 95% removal for all targeted contaminant except benzophenone |
• High degree microbial inactivation |
• Raised concerns on elevated AOCs and microbial regrowth potential after H2O2/ozone treatment and |
• Fluorescence excitation-emission matrix showed distinctively transformed organic matter footprints after treatment |
Standalone BAC |
DOC and nitrogen removal |
• Diminishing DOC removal rate after breakthrough is reached |
219
|
• More than 50% of total nitrogen removal rate |
Ozone/peroxide + RO |
General reuse applications |
• Ozone and ozone/peroxide showed similar trace organic contaminant removal performance, likely due to inherently high hydroxyl radicals in wastewater effluent |
220
|
• Formation of up to 48 ng L−1 NDMA is observed in wastewater effluent ozone systems, raising concern for future reuse applications |
Ultrafiltration + RO + UV |
Groundwater recharge |
• 13 out of 291 targeted compounds are detected in post-UV and post-RO water |
89
|
• Calculated risk quotient for detected chemicals indicates safe reuse |
ARGs, OPs, and other emerging microbial concerns
It is important to recognize the complexity of RWDSs as an ecological habitat and that microbial concerns reach beyond traditional indicator organism paradigms. Here we consider these emerging microbial concerns within a comprehensive microbiome/exposome framework. Several recent studies have utilized DNA sequencing to provide new insight into the composition of the drinking water microbiome, but few have attempted to characterize the recycled water microbiome. Recycled water, and even potable water, both represent surprisingly complex microbial niches, housing a vast array of microbial species about which little is known. Normal fecal indicator bacterial monitoring fails to provide information about the broader microbiome, particularly with respect to oligotrophic organisms residing in distribution systems. Thus, a more holistic approach for characterizing water quality is needed to accurately describe the water quality at the point of use. Here we elaborate on microbial aspects of the exposome that are generally unrecognized in the regulatory landscape and are particularly relevant to RWDSs. While occurrence of fecal-associated pathogens is also of importance in recycled water systems, we have limited the scope of this review to emerging microbial concerns.
Epidemiological studies examining associations between recycled water exposure and disease have been limited and are crucial to identifying potential for disease transmission, determining suitability for public use, and informing effective risk mitigation strategies. For example, Durand and Schwebach did not find an association of gastrointestinal illness when irrigating public parks with non-potable recycled water versus potable water (6% versus 7% of park users reporting symptoms associated with recycled wastewater irrigation versus potable water irrigation, respectively), though wet grass conditions during park usage were associated with an increased rate of illness.97 A study of food crop irrigation with recycled water over a five year period found no undesirable consequences to the quality of vegetables or soil, thus exposure restrictions for farm workers were not deemed necessary.98 In one study conducted in the U.S., even irrigation using trickling filter effluent wastewater was not associated with an increased rate of infection of rotavirus for residents of surrounding areas.99 A study that examined occurrence of methicillin resistant Staphylococcus aureus (MRSA) in spray irrigation workers using recycled water did not find the presence of the resistant organism in nasal swabs from any workers tested, though the odds of carrying a non-resistant strain of the organism were slightly higher among spray irrigation workers than among office workers.19 While isolated reports of disease stemming from exposure to recycled water are helpful, rigorous, long-term epidemiological studies are needed to more precisely determine sources of disease and accurately characterize risk and to address emerging concerns.
Of rising interest is the influence of the distinct physiochemical nature of recycled water on the regrowth or attenuation of emerging pathogens and contaminants, particularly considering exposures relevant to non-conventional water reuse applications. Especially when organic carbon is no longer a growth-limiting resource, conventional fecal bacterial indicators are likely to be even less relevant to shifts in microbial ecology during distribution and the associated health risk. Efforts are underway to recognize the importance of microbial ecological interactions in distribution systems and the potential to harness them to foster a distribution system that favors the growth of non-pathogenic bacteria over pathogenic ones.63 For example, Egli has identified the survival and growth strategies of various microbes in low-nutrient and stressed environments and competition between pathogens and the indigenous microbiota.100 A strategy of capitalizing upon specific ecological interactions, such as nutritional competition, antagonist growth, and symbiotic relationships for improved water quality and human health has been previously proposed for drinking water.101 This presents a potentially transformative and highly relevant approach for guiding RWDS management.
Opportunistic pathogens
RWDSs offer several unique characteristics that make them particularly well-suited for supporting regrowth of OPs. OPs in DWDSs are thought to be the primary source of waterborne disease in developed countries, including the U.S.102,103 Unlike most fecal pathogens, OPs do not typically impact the gastrointestinal system but rather they infect via alternative routes. To name a few, Legionella pneumophila, Acinetobacter baumanni, Mycobacterium avium, Burkholderia pseudomallei, Stenotrophomonas maltophilia, and Aspergillus fumigatus can infect hosts' lungs via inhalation;104–106S. aureus infects via broken skin or mucus membranes;107Pseudomonas aeruginosa can infect hosts via the bloodstream, eyes, ears, skin, or lungs;108 and Acanthamoeba spp. can cause infection of the eyes or central nervous system when inhaled or upon penetration of skin lesions.109 These alternative routes of infection make OPs of particular interest for recycled water, where exposure routes other than simple ingestion are more relevant. Inhalation of aerosols from cooling towers or spray irrigation and dermal contact with irrigated surfaces, are important routes of exposure that should be accounted for when considering risks associated with OPs in recycled water.
OPs possess several distinct properties that make them particularly well suited for growth in RWDSs (Fig. 3). OPs tend to be resistant to disinfection, ranging from 21–658 times as resistant to chlorine as Escherichia coli, as in the cases of P. aeruginosa and A. baumanii, respectively.110 Many OPs are also resistant to phagocytosis by amoebae, becoming enclosed within an amoebic cyst, where they can be further protected from disinfectants and other harsh environmental conditions. Biofilms, where OPs tend to reside, offer protection from similar environmental assaults, in addition to acidic and alkaline conditions and shear force from high flow velocities.101,110 OPs also tend to grow at low organic carbon concentrations, which is pertinent to both DWDSs and RWDSs.110 Stagnation is a notorious risk factor for OP outbreak, and is common in RWDSs due to intermittent demand and seasonal shutdown.111
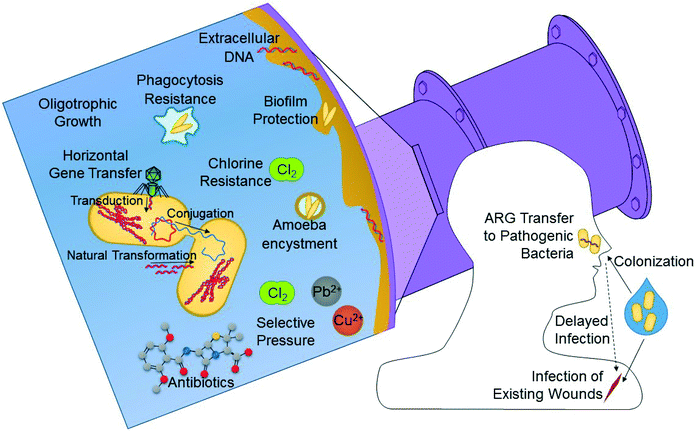 |
| Fig. 3 Processes by which antibiotic resistant bacteria and opportunistic pathogens (OPs) can re-grow in RWDSs and relevant exposure routes. | |
Antibiotic resistance genes
Antibiotic resistance among human pathogens is a major public health concern. In the U.S., the Centers for Disease Control has estimated that antibiotic resistant bacteria cause at least two million infections and 23
000 deaths each year.112 ARGs are now well-known to be elevated in human-contaminated surface waters,113–116 however with respect to human pathogens, specifically, there is reasonable evidence that they can gain ARGs from environmental bacteria via horizontal gene transfer (Fig. 3).117,118 Therefore, all members of the microbiome carrying ARGs are potentially of concern, particularly those that are common in human pathogens. In addition to the possibility of infection by antibiotic resistant bacteria upon exposure, human hosts may also become colonized and infected later.119–121 Similarly, it is possible that horizontal gene transfer may occur from colonized non-pathogenic bacteria to pathogenic ones, leading to antibiotic resistant infection. Thus, infection by antibiotic resistant bacteria may occur at a time and place separate from that of the initial exposure, which complicates traditional dose–response risk assumptions. ARGs or bacteria expressing ARGs corresponding to resistance to aminoglycoside, beta-lactam, chloramphenicol, fluoroquinolone, lincomycide, linezolid, lipopeptide, macrolide, sulfonamide, tetracyclines, and vancomycin antibiotics have been previously identified in recycled water or environments directly impacted by irrigation, infiltration, or groundwater recharge using recycled water.122–128 Since antibiotic resistance is a natural phenomenon inherent among many bacteria, studies that compare these abundances to relevant control environments, such as corresponding potable water or environments unimpacted by recycled water are of particular value. While the nature of reusing human wastewater means that prior to treatment, human pathogens or other bacteria carrying ARGs will be enriched compared to other source waters, multiple studies have demonstrated that ARGs are often not removed during treatment, and in some cases, are even amplified.129–132 Additionally, a study by Fahrenfeld et al. found that ARGs may also increase during distribution of recycled water as a broader range of monitored ARGs were present in point of use samples than in samples leaving the treatment plant.122
Various features of recycled water potentially make it a prime medium for the growth of antibiotic resistant bacteria and propagation of their associated ARGs during distribution. In particular, residual antibiotics that escape removal during treatment can exert selective pressure and encourage persistence of ARG-carrying bacteria. Though antibiotics will likely be found in recycled water at sub-lethal concentrations, this low level exposure has actually been shown to encourage the persistence of bacteria that carry ARGs via several mechanisms.133–135 Gullberg et al. found that bacteria maintained plasmids carrying beta-lactam resistance genes even at concentrations of antibiotics and heavy metals nearly 140 times below the compound's minimum inhibitory concentration.136 Other studies have also demonstrated that sublethal antibiotics can stimulate propagation of ARGs by activating horizontal gene transfer.137–142 Prudhomme et al. demonstrated that intermediate concentrations of streptomycin induced genetic transformation in Streptococcus pneumoniae.140 Beaber et al. demonstrated that ‘SOS response’ among Vibrio cholerae induced by the presence of ciprofloxacin enhances transfer of resistance genes via conjugation.142 Low levels of antibiotics or other selective agents also act to encourage adaptive evolution including development of resistance mutants.135
Antibiotics are not the only antimicrobials with potential to select for ARGs in recycled water systems. Heavy metals, such as copper and iron (which are commonly used in distribution systems), have long been suspected to select for ARGs in a variety of environments.143 Metal-driven selection of ARGs is also of concern due to the presence of various heavy metals capable of ARG selection common in many wastewaters, such as copper, zinc, nickel, mercury, and even nanosilver.144,145 Disinfectants have also been known to select for ARGs.146–148 Following chlorination, E. coli carrying the tetA tetracycline resistance gene were found to be even more tolerant to tetracycline than non-chlorinated E. coli.148 Chlorination has also been reported to concentrate a variety of ARGs in potable water.147
In addition to increasing ARGs via mutations, natural selection, and horizontal gene transfer, presence of residual antibiotics can enhance biofilm formation.149 Studies of Staphylococcus aureus, E. coli, and P. aeruginosa have all indicated that sub-inhibitory concentrations of various antibiotics induce biofilm formation.150–152 Extensive biofilm formation provides a fertile environment for the transfer of ARGs via horizontal gene transfer. Dense microbial communities existing in biofilms with extensive cell to cell contact facilitate transfer via conjugation.153,154 Notably, a key component of biofilms, extracellular polymeric substances, is partially comprised of DNA expelled from cells.155 This may provide a reservoir of free DNA-based ARGs, which have been shown to be available for uptake into cells via transformation.156,157 Biofilms themselves offer protection from antibiotics or other antimicrobial agents via the principle of collective resistance, where cells are physically shielded from exposure to the antimicrobial.158
While transmission of antibiotic resistant bacteria is an acute public health threat, the possibility that water reuse may exacerbate the overall spread of antibiotic resistance has been suggested.159 The water cycle as a whole has recently been subject to scrutiny as a potentially important, yet understudied, route for the spread of antibiotic resistance.160–162 Given the gravity of the antibiotic resistance problem and several lines of reasoning that water reuse can contribute to its spread, additional research is urgently needed to determine whether consideration of antibiotic resistance should be of central concern to comprehensive long-term risk management strategies.
Viruses
Though removal of viruses in recycled water is of great importance, the presence of viruses in recycled water is rarely monitored. Treatment goals and regulations regarding virus removal are typically presented in the form of expected log-removal achieved through treatment such as disinfection, largely due to the analytical difficulty of direct virus detection.6 Low recovery rates, complex and time-consuming laboratory culture procedures, slow turn-around time for culture results, and inability of molecular techniques to differentiate viable from non-viable viruses are major challenges. Problems with the indirect monitoring paradigm may arise, however, because viruses may be resistant to some modes of disinfection. For example, adenoviruses are known to resist ultraviolet irradiation.6
A recent study of the viral metagenome (i.e., total DNA extracted from viral component) revealed approximately 108 virus-like particles (VLP) per mL in non-potable recycled water, 1000 times more than that measured in potable water.163 Further, genetic markers corresponding to viruses targeting eukaryotes were non-detectable in potable water, while two percent of the viruses in recycled water corresponded to eukaryotic hosts. This is logical, indicating that recycled water is more susceptible to carrying viruses associated with humans than traditional potable water.
Bacteriophages, which represent the vast majority of viruses in both potable and recycled waters, have their own relevance to human health as they act as agents of transfer for ARGs among bacteria via transduction. Bacteriophages have been largely neglected as constituents in potable and recycled water, though they have been found to be highly abundant in both raw wastewater (108 VLP mL−1) and in potable water (105–106 VLP mL−1).163 Though transduction is generally considered a rare transfer event, occurring only once in every 107–109 phage infections,164 the shear abundance of bacteriophages documented in wastewater and potable water suggests that it is likely a significant phenomenon in recycled water.
Amoebae
FLA are of growing concern in drinking water plumbing. Many FLA, such as Acanthamoeba spp. and Vermameoba spp., graze on bacterial biofilms, and in doing so, can serve as an important vector for amplifying and disseminating OPs.165 For example, Legionella, Mycobacterium, and Pseudomonas spp. can amplify within FLA when grazed upon, which enhances their dissemination and virulence.165
FLA themselves can sometimes be pathogenic, as is the case with keratitis or primary amoebic meningoencephalitis (PAM).165 Similar to other OPs, non-ingestion routes of exposure are important for pathogenic FLA. PAM is contracted when N. fowleri is forced into the nasal cavity and migrates to the brain, while keratitis occurs when pathogenic Acanthamoeba spp. infect the eye.166 Such exposures have been documented both in recreational and drinking water.109,167,168 However, relevant to recycled water, inhalation is under investigation as a primary transmission method.168,169
The design of RWDSs can instigate the growth of biofilm, providing a reservoir for FLA and an environment to promote interactions with amoeba-resisting bacteria. Recycled waters have complex microbial communities and high availability of nutrients and other organic matter, creating optimal conditions for biofilm establishment.170 Recent studies have also shown increased chlorine resistance of FLA in the presence of naturally established biofilm171,172 and even non-biofilm Vermamoeba spp. have been observed to resist chlorination.173
The relationship between L. pneumophila and FLA has been the most closely studied. Resistance to amoebae can provide protection from disinfection, competition, environmental stress and predation for L. pneumophila.174–176 Additionally, different FLA have been shown to survive a wide range of temperatures, from 10–45 °C, with some cases indicating survival near 0 °C, potentially allowing for protection of Legionella spp. and other amoeba-resisting bacteria during winter, while amoeba are encysted.165,177–179 Little is still known about the diversity and abundance of amoebae and their interactions with amoeba-resisting bacteria in drinking water, let alone recycled water. Gaining a better understanding of the interactions between these microorganisms and the ways in which this may aid the growth of pathogenic bacteria is essential for better understanding the exposome associated with recycled water.
Algae
Algae are a common nuisance in recycled water systems. Though algal growth frequently occurs in systems that use open storage rather than in distribution system pipes, algal cells can persist throughout distribution systems where they have been found to correlate with AOC and BDOC.29 Decaying algal cells can even be a source of BDOC, contributing to the regrowth of other microbial constituents. Increased regrowth resulting from organic carbon made available from decaying algal cells has also been linked with a loss of oxygen and dissipation of chlorine residual.29 Elevated concentrations of algae may carry potential for the production of harmful algal toxins. Cyanobacteria toxins have been linked to liver damage, neurotoxicity, gastroenteritis, pneumonia, and even death.180 Though these symptoms primarily arise from ingestion of the toxins, skin irritations and allergic reactions have been noted following dermal contact with cyanobacteria toxins and respiratory disease has been documented following suspected inhalation of the toxins.181 In cases where non-potable reuse occurs, these problems may be particularly challenging to identify as taste and odor complaints from consumers will be unlikely.
Conclusion
Given the increasing trend of water reuse across the globe, it is important that all aspects, including end-users, treatment plant management and operation, regulation, and public health protection are taken into consideration in the planning and implementation of water reuse risk management strategies. In this paper, we summarized the inherently different biochemistry of recycled water in the distribution system as a function of various usage and operational factors. We also discussed acute and long-term risks from the chemical and microbial contaminants that may result from multi-dimensional usage routes and the associated exposure risks associated with various end use of the recycled waters.
Increased awareness of traditionally underappreciated routes of exposure is key to the safe use of recycled water. The history of drinking water epidemiology provides numerous examples of infection via atypical routes of exposure. For example, in 2015 an outbreak of Legionnaire's disease that killed 12 people in New York City was linked to infection via aerosolized bacteria from cooling towers sourced from potable drinking water.182N. fowleri infection from drinking water has occurred from use for nasal irrigation and in children via bathing or playing on an outdoor water slide.168 Prevention of infection as a result of unintended exposures with recycled water requires proactive action when planning for treatment and distribution of recycled water. One key “critical control point” that must be considered in this planning is the distribution system to avoid degradation of water quality during distribution. Treating recycled water to remove nutrients and achieve biostability is one promising approach to help ensure safe water at the point of use, but additional treatment at the point of use may also be necessary in some cases, as both the physiochemical and microbial water quality change significantly during distribution and in premise plumbing systems. A key research gap exists regarding the most effective approaches for achieving biostability of recycled water during distribution. Specific and cost-effective engineering controls for nutrient recycling and limiting regrowth during distribution must be identified for respective intended uses. Identification of emerging chronic contaminants and microbial contaminants is also important in minimizing potentially harmful exposures. Rigorous studies that examine the health implications of non-traditional routes of exposure are quite limited and are challenging to design given the lack of available knowledge about infectious doses (particularly based on non-oral routes of exposure), magnitude of exposure via non-traditional routes, and concentrations of emerging contaminants that are typical of recycled water. In addition, virulence and individual susceptibility varies widely for many of the microbial constituents discussed, making it important to consider exposure of immunocompromised populations when assessing risk. In addition to these research gaps, development of quantitative microbiological risk assessments (QMRA) would be extremely valuable for assessing the risk associated with the presence OPs, ARGs, FLA, and viruses in recycled water. Epidemiological studies are also critical for linking actual human illness and associated microbial sources with recycled water. Finally, research is needed to tailor treatment processes to serve specific intended end uses (e.g.Table 1), along with addressing emerging concerns identified here, while also developing best management practices for distribution systems and premise plumbing for preventing re-growth and deterioration of water quality in RWDSs.
While overarching regulations that consider the comprehensive implications and scope of water recycling are currently lacking in many places, there are also practical lessons we can learn from international leaders on adopting a comprehensive risk management approach towards water reuse, notably the Australian Guidelines for Water Recycling, the WHO's framework of WSP, and the HACCP paradigm. Complementing these strategies with a holistic approach focused on the human exposome creates a framework in which consideration of user exposures drives establishment of water quality standards. Though a regulatory framework that addresses the exposure risks and potential for regrowth associated with use of recycled water is an ideal long term goal, an interim approach of more basic best management practices, as suggested by Jjemba et al., are a reasonable starting place to enable municipalities utilizing recycled water to proactively act to limit bacterial regrowth and preserve water quality at the point of use.29 Best management practices should continually be revised as knowledge gaps are addressed, to ensure that the most meaningful water quality indicators are targeted.
Adoption of the human exposome paradigm aims to ensure comprehensive understanding of the risks and uncertainties regarding alternative recycled water sources. Enhanced knowledge could provide critical guidance on safe management and inform much-needed regulations as the use of recycled water expands. However, while this exposome approach highlights the multi-dimensional risks and uncertainties regarding use of recycled water, it also must be recognized that water reuse plays an integral role in addressing the grand challenge of water scarcity. It is estimated that a third of the world's population is currently living with moderate to high levels of water stress183 and approximately 50% will suffer water shortages by 2025.184 Implementing water reuse projects is imperative to meet water needs in drought-stricken areas, despite potential risks and concerns. As estimated by Brown, current groundwater sources, serving more than half of the world's population, are largely overdrafted.185 Lack of new alternative water supplies, compounded with increasing water demand, would further intensify water scarcity stress. Schoreder et al. has estimated that the potential benefits of reuse could offset water supply for a community of 1 million people by 75 million gallons per day.186 In cases where water scarcity lends to the likelihood of de facto reuse, then it is better to have intentional reuse guided by best management practices to minimize risks.
Equally important to the exposure risk from recycled water is lack of access to traditional potable water sources and poor water quality due to degraded source water. Globally, there are over five million deaths associated with poor water quality every year.187 Achieving an environmentally sustainable and socially beneficially water demand management plan requires proactive evaluation of the highest priority needs and identification of the key drivers and barriers to the implementation of water recycling projects. Positive associations between information availability and the acceptance of water reuse have been noted.188–190 As end users become more educated about this alternative water source, their willingness to use recycled water increases.191 Nonetheless, comprehensively addressing all possible public health concerns will be an essential pillar to advancing water sustainability.
Glossary
AOC | Assimilable organic carbon |
ARG | Antibiotic resistance gene |
BAC | Biological activated carbon |
BDOC | Biodegradable dissolved organic carbon |
CAS | Conventional activated sludge |
CFU | Colony forming unit |
DBP | Disinfection byproduct |
DOC | Dissolved organic carbon |
DOM | Dissolved organic matter |
DON | Dissolved organic nitrogen |
DPR | Direct potable reuse |
DWDS | Drinking water distribution system |
FLA | Free-living amoebae |
HACCP | Hazard analysis and critical control point |
MRSA | Methicillin resistant Staphylococcus aureus |
NOM | Natural organic matter |
OP | Opportunistic pathogen |
PAM | Primary amoebic meningoencephalitis |
QMRA | Quantitative microbiological risk assessment |
RO | Reverse osmosis |
RWDS | Recycled water distribution system |
THAAFP | Total haloacetic acid formation potential |
TTHM | Total trihalomethanes |
TTHMFP | Total trihalomethane formation potential |
UV | Ultraviolet irradiation |
VLP | Virus-like particle |
WHO | World Health Organization |
WSP | Water safety plan |
WWTP | Wastewater treatment plant |
Acknowledgements
This work was supported by The Alfred P. Sloan Foundation Microbiology of the Built Environment Program, the National Science Foundation Award # 1438328, the Water Environment Research Foundation Paul L. Busch Award, and Graduate Research Fellowship Program Grant # DGE 0822220. We would like to thank Owen Strom for assistance creating figures.
References
- J. Anderson, Water Sci. Technol.: Water Supply, 2003, 3, 1–10 CAS.
- D. Goodwin, M. Raffin, P. Jeffrey and H. M. Smith, Environ. Sci.: Water Res. Technol., 2015, 1, 709–722 Search PubMed.
- D. Gerrity, B. Pecson, R. S. Trussell and R. R. Trussell, J. Water Supply: Res. Technol.--AQUA, 2013, 62, 321–338 CrossRef CAS.
- P. Jjemba, W. Johnson, Z. Bukhari and M. LeChevallier, J. Water Reuse Desalin., 2014, 4, 209 CrossRef CAS.
-
F. Digiano and M. Aitkin, Workshop on Water Reuse and Dual Systems for Potable and Non-Potable Water Supply. U.S. Environmental Protection Agency, Final Report for Cooperative Agreement: 83422201-0, 2010, 51 pp.
-
USEPA, Guidelines for Water Reuse, United States Environmental Protection Agency, 2012 Search PubMed.
- NRMMC EPHC & AHMC, Australian Guidelines for Water Recycling: Managing Health and Environmental Risks (Phase 1), Natural Resource Ministerial Management Council, Environment Protection and Heritage Council and Australian Health Ministers, 2006.
- J. Lahnsteiner and G. Lempert, Water Sci. Technol., 2007, 55, 441–448 CrossRef CAS PubMed.
- M. Agulló-Barceló, F. Oliva and F. Lucena, Environ. Sci. Pollut. Res., 2013, 20, 4448–4454 CrossRef PubMed.
- V. J. Harwood, A. D. Levine, T. M. Scott, V. Chivukula, J. Lukasik, S. R. Farrah and J. B. Rose, Appl. Environ. Microbiol., 2005, 71, 3163–3170 CrossRef CAS PubMed.
-
J. Swierc, D. Page, J. Van Leeuwen and P. J. Dillon, CSIRO L. Water Tech. Rep., 2005, vol. 20 Search PubMed.
-
J. Bartram, L. Corrales, A. Davison, D. Deere, D. Drury, B. Gordon, G. Howard, A. Rinehold and M. Stevens, Water safety plan manual: step-by-step risk management for drinking-water suppliers, World Health Organization, Geneva, 2009 Search PubMed.
- G. W. Miller and D. P. Jones, Toxicol. Sci., 2014, 137, 1–2 CrossRef CAS PubMed.
- C. P. Wild, Cancer Epidemiol., Biomarkers Prev., 2005, 14, 1847–1850 CAS.
- J. Rice, A. Wutich and P. Westerhoff, Environ. Sci. Technol., 2013, 47, 11099–11105 CrossRef CAS PubMed.
- M. Lafforgue and V. Lenouvel, Environ. Sci.: Water Res. Technol., 2015, 1, 622–631 Search PubMed.
-
J. Sutherland, E. Steinle-Darling, A. Salveson and E. Mancha, presented in part at Water Quality Technology Conference, Fort Worth, July, 2015 Search PubMed.
- D. G. Addiss, J. P. Davis, M. LaVenture, P. J. Wand, M. A. Hutchinson and R. M. McKinney, Am. J. Epidemiol., 1989, 130, 557–568 CAS.
- R. E. Rosenberg Goldstein, S. a Micallef, S. G. Gibbs, X. He, A. George, A. A. R. Sapkota, S. W. Joseph and A. A. R. Sapkota, Int. J. Environ. Res. Public Health, 2014, 11, 4340–4355 CrossRef CAS PubMed.
- F. Brissaud, E. Blin, S. Hemous and L. Garrelly, Water Sci. Technol., 2008, 57, 781–787 CrossRef CAS PubMed.
- K. E. Borboudaki, N. V. Paranychianakis and K. P. Tsagarakis, Environ. Manage., 2005, 36, 610–623 CrossRef CAS PubMed.
- C. P. Gerba, C. Wallis and J. L. Melnick, Appl. Microbiol., 1975, 30, 229–237 CAS.
- W. G. Hlady, R. C. Mullen, C. S. Mintz, B. G. Shelton, R. S. Hopkins and G. L. Daikos, Am. J. Epidemiol., 1993, 138, 555–562 CAS.
- T. E. Haupt, R. T. Heffernan, J. J. Kazmierczak, H. Nehls-Lowe, B. Rheineck, C. Powell, K. K. Leonhardt, A. S. Chitnis and J. P. Davis, Infect. Control Hosp. Epidemiol., 2012, 33, 185–191 CrossRef PubMed.
- T. N. Palmore, F. Stock, M. White, M. Bordner, A. Michelin, J. E. Bennett, P. R. Murray and D. K. Henderson, Infect Control Hosp Epidemiol, 2009, 30, 764–768 CrossRef PubMed.
- J. B. Rose and C. S. Clark, Crit. Rev. Environ. Control, 1986, 16, 231–256 CrossRef.
-
ASCE 2013 Report Card for America's Infrastructure, http://infrastructurereportcard.org/a/#p/home, (accessed December 2015) Search PubMed.
- H. Wang, C. Hu, X. Hu, M. Yang and J. Qu, Water Res., 2012, 46, 1070–1078 CrossRef CAS PubMed.
- P. K. Jjemba, L. a. Weinrich, W. Cheng, E. Giraldo and M. W. LeChevallier, Appl. Environ. Microbiol., 2010, 76, 4169–4178 CrossRef CAS PubMed.
- P. Sarin, V. L. Snoeyink, J. Bebee, K. K. Jim, M. A. Beckett, W. M. Kriven and J. A. Clement, Water Res., 2004, 38, 1259–1269 CrossRef CAS PubMed.
-
USEPA, http://archive.epa.gov/region03/dclead/web/html/index-3.html (accesed January 2016) Search PubMed.
-
Mlive, http://www.mlive.com/news/flint/index.ssf/2015/09/new_testing_shows_flint_water.html (accessed January 2016).
-
MATCOR, http://www.matcor.com/water-line-break-corrosion-a-headache-for-tucson-water/ (accessed January 2016).
- F. Yang, B. Shi, Y. Bai, H. Sun, D. a. Lytle and D. Wang, Water Res., 2014, 59, 46–57 CrossRef CAS PubMed.
- M. Tang, S. Triantafyllidou and M. A. Edwards, Environ. Sci. Technol., 2015, 49, 8697–8703 CrossRef CAS PubMed.
-
M. V. Story and C. E. Kaucner, Water Quality Research Australia: Research Report 79, 2009, p. 122 Search PubMed.
- H. Rufenacht and H. Guibentif, J. Water Supply: Res. Technol.--AQUA, 1997, 46, 196–201 Search PubMed.
- R. M. Willis, R. A. Stewart, P. R. Williams, C. H. Hacker, S. C. Emmonds and G. Capati, Desalination, 2011, 272, 201–211 CrossRef CAS.
- J. Nawrocki, U. Raczyk-Stanisławiak, J. Świetlik, A. Olejnik and M. J. Sroka, Water Res., 2010, 44, 1863–1872 CrossRef CAS PubMed.
- P. Ji, J. Parks, M. A. Edwards and A. Pruden, PLoS One, 2015, 10, e0141087 Search PubMed.
- W. J. Rhoads, A. Pruden and M. A. Edwards, Environ. Sci.: Water Res. Technol., 2016, 2, 164–173 CAS.
- H.-Y. Hu, Y. Du, Q.-Y. Wu, X. Zhao, X. Tang and Z. Chen, Sci. Total Environ., 2016, 551-552, 133–142 CrossRef CAS PubMed.
- H. K. Shon, S. Vigneswaran and S. A. Snyder, Crit. Rev. Environ. Sci. Technol., 2006, 36, 327–374 CrossRef CAS.
- B. Rittman and V. Snoeyink, J. - Am. Water Works Assoc., 1984, 76, 106–114 Search PubMed.
- P. W. J. J. van der Wielen and D. van der Kooij, Water Res., 2010, 44, 4860–4867 CrossRef CAS PubMed.
- P. Thayanukul, F. Kurisu, I. Kasuga and H. Furumai, Water Res., 2013, 47, 225–232 CrossRef CAS PubMed.
- L. A. Weinrich, P. K. Jjemba, E. Giraldo and M. W. LeChevallier, Water Res., 2010, 44, 5367–5375 CrossRef CAS PubMed.
-
M. Karim and M. W. LeChevallier, Microbiological quality of reuse water, Internal Report, Voorhees, NJ, 2005 Search PubMed.
- M. W. LeChevallier, N. J. Welsh and D. B. Smith, Appl. Environ. Microbiol., 1996, 62, 2201–2221 CAS.
- X. Zhao, H. Hu, S. Liu, F. Jiang, X. Shi, M. Li and X. Xu, Front. Environ. Sci. Eng., 2013, 7, 483–491 CrossRef CAS.
- E. Khan, R. W. Babcock Jr., I. H. Suffet and M. K. Stenstrom, Water Environ. Res., 1998, 70, 1025–1032 CrossRef CAS.
- L. S. McNeill and M. Edwards, J. - Am. Water Works Assoc., 2014, 93, 88–100 Search PubMed.
- M. Edwards and N. Sprague, Corros. Sci., 2001, 43, 1–18 CrossRef CAS.
- A. K. Camper, Int. J. Food Microbiol., 2004, 92, 355–364 CrossRef CAS PubMed.
- M. W. LeChevallier, C. D. Lowry, R. G. Lee and D. L. Gibbon, J. - Am. Water Works Assoc., 1993, 85, 111–123 CAS.
- H. Wang, C. Hu, L. Zhang, X. Li, Y. Zhang and M. Yang, Water Res., 2014, 65, 362–370 CrossRef CAS PubMed.
- Y. Zhu, H. Wang, X. Li, C. Hu, M. Yang and J. Qu, Water Res., 2014, 60, 174–181 CrossRef CAS PubMed.
-
A. K. Camper and P. Dircks, Distribution system as reactor, 1996, https://www.biofilm.montana.edu/resources/images/industrial-systems-processes/distribution-system-reactor.html, (accessed January 2016) Search PubMed.
- S. T. Powell, J. - Am. Water Works Assoc., 1921, 8, 522–529 CAS.
- A. M. Buswell, J. - Am. Water Works Assoc., 1938, 30, 1651–1654 CAS.
- N. J. Howard, J. - Am. Water Works Assoc., 1940, 32, 1501–1506 CAS.
- J. Hall, A. D. Zaffiro, R. B. Marx, P. C. Kefauver, E. R. Krishnan, R. C. Haught and J. G. Herrmann, J. - Am. Water Works Assoc., 2007, 99, 66–77 CAS.
- D. Berry, C. Xi and L. Raskin, Curr. Opin. Biotechnol., 2006, 17, 297–302 CrossRef CAS PubMed.
- P. Piriou, B. E. Rittmann and J. E. Woolschlager, Urban Water J., 2005, 2, 69–79 CrossRef.
- S. Masters, H. Wang, A. Pruden and M. A. Edwards, Water Res., 2015, 68, 140–149 CrossRef CAS PubMed.
- O. M. Ajibode, C. Rock, K. Bright, J. E. T. Mclain, C. P. Gerba and I. L. Pepper, J. Water Reuse Desalin., 2013, 3, 185–196 CrossRef CAS.
- M. K. Ramseier, A. Peter, J. Traber and U. von Gunten, Water Res., 2011, 45, 2002–2010 CrossRef CAS PubMed.
- K. A. Steed and J. O. Falkinham, Appl. Environ. Microbiol., 2006, 72, 4007–4011 CrossRef CAS PubMed.
- R. H. Taylor, J. O. Falkinham, C. D. Norton and M. W. LeChevallier, Appl. Environ. Microbiol., 2000, 66, 1702–1705 CrossRef CAS PubMed.
- I. Marchesi, P. Marchegiano, A. Bargellini, S. Cencetti, G. Frezza, M. Miselli and P. Borella, J. Hosp. Infect., 2011, 77, 47–51 CrossRef CAS PubMed.
- M. Dupuy, S. Mazoua, F. Berne, C. Bodet, N. Garrec, P. Herbelin, F. Ménard-Szczebara, S. Oberti, M.-H. Rodier, S. Soreau, F. Wallet and Y. Héchard, Water Res., 2011, 45, 1087–1094 CrossRef CAS PubMed.
- J.-J. Huang, H.-Y. Hu, F. Tang, Y. Li, S.-Q. Lu and Y. Lu, Water Res., 2011, 45, 2775–2781 CrossRef CAS PubMed.
- M. Guo, J. Huang, H. Hu and W. Liu, Biomed. Environ. Sci., 2011, 24, 400–407 Search PubMed.
- J. Süss, S. Volz, U. Obst and T. Schwartz, Water Res., 2009, 43, 3705–3716 CrossRef PubMed.
- R. M. Clark and M. Sivaganesan, J. Environ. Eng., 1998, 124, 1203–1210 CrossRef CAS.
- S. W. Krasner, Philos. Trans. R. Soc., A, 2009, 367, 4077–4095 CrossRef CAS PubMed.
- T. Sirivedhin and K. A. Gray, Water Res., 2005, 39, 1025–1036 CrossRef CAS PubMed.
- C. Nurizzo, M. Antonelli, M. Profaizer and L. Romele, Desalination, 2005, 176, 241–253 CrossRef CAS.
- C. C. Wang, Z. G. Niu and Y. Zhang, J. Hazard. Mater., 2013, 262, 179–188 CrossRef CAS PubMed.
- C. M. Coetsier, S. Spinelli, L. Lin, B. Roig and E. Touraud, Environ. Int., 2009, 35, 787–792 CrossRef CAS PubMed.
- S. Mompelat, B. Le Bot and O. Thomas, Environ. Int., 2009, 35, 803–814 CrossRef CAS PubMed.
- M. Gros, M. Petrović, A. Ginebreda and D. Barceló, Environ. Int., 2010, 36, 15–26 CrossRef CAS PubMed.
- A. Jelic, M. Gros, A. Ginebreda, R. Cespedes-Sánchez, F. Ventura, M. Petrovic and D. Barcelo, Water Res., 2011, 45, 1165–1176 CrossRef CAS PubMed.
- M. Huerta-Fontela, M. T. Galceran and F. Ventura, Water Res., 2011, 45, 1432–1442 CrossRef CAS PubMed.
- Y. Ma, M. Li, M. Wu, Z. Li and X. Liu, Sci. Total Environ., 2015, 518–519, 498–506 CrossRef CAS PubMed.
- D. W. Kolpin, M. Skopec, M. T. Meyer, E. T. Furlong and S. D. Zaugg, Sci. Total Environ., 2004, 328, 119–130 CrossRef CAS PubMed.
- G. A. Loraine and M. E. Pettigrove, Environ. Sci. Technol., 2006, 40, 687–695 CrossRef CAS PubMed.
- J. D. Peterson, R. R. Murphy, Y. Jin, L. Wang, M. B. Nessl and K. Ikehata, Water Environ. Res., 2011, 83, 1853–1875 CrossRef CAS.
- F. Busetti, M. Ruff and K. L. Linge, Environ. Sci.: Water Res. Technol., 2015, 1, 659–667 CAS.
- S. Weber, S. Khan and J. Hollender, Desalination, 2006, 187, 53–64 CrossRef CAS.
-
S. Khan, in Sustainable Water, ed. R. Hester and R. Harrison, The Royal Society of Chemistry, London, 2011, ch. 6, pp. 114–137 Search PubMed.
- M. M. Huber, S. Canonica, G. Y. Park and U. Von Gunten, Environ. Sci. Technol., 2003, 37, 1016–1024 CrossRef CAS PubMed.
- I. H. Kim, N. Yamashita, Y. Kato and H. Tanaka, Water Sci. Technol., 2009, 59, 945–955 CrossRef CAS PubMed.
- L. H. M. L. M. Santos, A. N. Araújo, A. Fachini, A. Pena, C. Delerue-Matos and M. C. B. S. M. Montenegro, J. Hazard. Mater., 2010, 175, 45–95 CrossRef CAS PubMed.
- C. R. Tyler, S. Jobling and J. P. Sumpter, Crit. Rev. Toxicol., 2008, 28, 319–361 CrossRef PubMed.
- D. W. Kolpin, E. T. Furlong, M. T. Meyer, E. M. Thurman, S. D. Zaugg, L. B. Barber and H. T. Buxton, Environ. Sci. Technol., 2002, 36, 1202–1211 CrossRef CAS PubMed.
- R. Durand and G. Schwebach, Am. J. Public Health, 1989, 79, 1659–1660 CrossRef CAS PubMed.
- B. Sheikh, R. P. Cort, W. R. Kirkpatrick, R. S. Jaques and T. Asano, Res. J. Water Pollut. Control Fed., 1990, 62, 216–226 CAS.
- R. L. Ward, D. R. Knowlton, J. Stober, W. Jakubowski, T. Mills, P. Graham and D. E. Camann, Water Res., 1989, 23, 1503–1509 CrossRef.
- T. Egli, Water Res., 2010, 44, 4826–4837 CrossRef CAS PubMed.
- H. Wang, M. A. Edwards, J. O. Falkinham 3rd and A. Pruden, Environ. Sci. Technol., 2013, 47, 10117–10128 CrossRef CAS PubMed.
-
A. Pruden, M. A. Edwards, J. O. Falkinham III, M. Arduino, J. Bird, R. Birdnow, E. Bedard, A. Camper, J. Clancy, E. Hilborn, V. Hill, A. Martin, S. Masters, N. R. Pace, M. Prevost, A. Rosenblatt, W. Rhoads, J. E. Stout and Y. Zhang, State of the Science and Research Needs for Opportunistics Pathogens in Premise Plumbing: Methodology, microbial ecology, and epidemiology, Water Research Foundation, Denver, CO, 2013 Search PubMed.
- J. M. Brunkard, E. Ailes, V. A. Roberts, V. Hill, E. D. Hilborn, G. F. Craun, A. Rajasingham, A. Kahler, L. Garrison, L. Hicks, J. Carpenter, T. J. Wade, M. J. Beach and J. S. Yoder, MMWR Morb Mortal Wkly Rep., 2008, 57, 1–38 Search PubMed.
- D. W. Fraser, T. R. Tsai, W. Orenstein and W. E. Parkin, N. Engl. J. Med., 1989, 297, 1189–1197 CrossRef PubMed.
- A. Y. Peleg, H. Seifert and D. L. Paterson, Clin. Microbiol. Rev., 2008, 21, 538–582 CrossRef CAS PubMed.
- C. R. Horsburgh, N. Engl. J. Med., 1991, 324, 1332–1338 CrossRef PubMed.
- F. D. Lowy, N. Engl. J. Med., 1998, 339, 520–532 CrossRef CAS PubMed.
- G. P. Bodey, R. Bolivar, V. Fainstein and L. Jadeja, Rev. Infect. Dis., 1983, 5, 279–313 CrossRef CAS PubMed.
- F. Marciano-Cabral and G. Cabral, Clin. Microbiol. Rev., 2003, 16, 273–307 CrossRef PubMed.
- J. Falkinham, Int. J. Environ. Res. Public Health, 2015, 12, 4533–4545 CrossRef PubMed.
- H. Wang, M. Edwards, J. O. Falkinham and A. Pruden, Appl. Environ. Microbiol., 2012, 78, 6285–6294 CrossRef CAS PubMed.
- US CDC, Antibiotic Resistance Threats in the United States, 2013 Search PubMed.
- A. Pruden, M. Arabi and H. N. Storteboom, Environ. Sci. Technol., 2012, 46, 11541–11549 CrossRef CAS PubMed.
- D. W. Graham, S. Olivares-Rieumont, C. W. Knapp, L. Lima, D. Werner and E. Bowen, Environ. Sci. Technol., 2011, 45, 418–424 CrossRef CAS PubMed.
- G. C. A. Amos, P. M. Hawkey, W. H. Gaze and E. M. Wellington, J. Antimicrob. Chemother., 2014, 69, 1785–1791 CrossRef CAS PubMed.
- E. Marti, J. Jofre and J. L. Balcazar, PLoS One, 2013, 8, e78906 CAS.
- R. Benveniste and J. Davies, Proc. Natl. Acad. Sci. U. S. A., 1973, 70, 2276–2280 CrossRef CAS.
- K. J. Forsberg, A. Reyes, B. Wang, E. M. Selleck, M. O. A. Sommer and G. Dantas, Science, 2012, 337, 1107–1111 CrossRef CAS PubMed.
- K. A. Davis, J. J. Stewart, H. K. Crouch, C. E. Florez and D. R. Hospenthal, Clin. Infect. Dis., 2004, 39, 776–782 CrossRef PubMed.
- C. von Eiff, K. Becker, K. Machka, H. Stammer and G. Peters, N. Engl. J. Med., 2001, 344, 11–16 CrossRef CAS PubMed.
- D. Bogaert, R. De Groot and P. W. M. Hermans, Lancet Infect. Dis., 2004, 4, 144–154 CrossRef CAS PubMed.
- N. L. Fahrenfeld, Y. J. Ma, M. O'Brien and A. Pruden, Front. Microbiol., 2013, 4, 1–10 Search PubMed.
- F. Barker-Reid, E. M. Fox and R. Faggian, J. Water Health, 2010, 8, 521–531 CrossRef CAS PubMed.
- J. McLain and C. Williams, Sustainability, 2014, 6, 1313–1327 CrossRef CAS.
- R. E. R. Goldstein, S. A. Micallef, S. G. Gibbs, A. George, E. Claye, A. Sapkota, S. W. Joseph and A. R. Sapkota, Sci. Total Environ., 2014, 466, 404–411 CrossRef PubMed.
- F. H. Wang, M. Qiao, Z. E. Lv, G. X. Guo, Y. Jia, Y. H. Su and Y. G. Zhu, Environ. Pollut., 2014, 184, 247–253 CrossRef CAS PubMed.
- F. Wang, M. Qiao, J. Su, Z. Chen, X. Zhou and Y. Zhu, Environ. Sci. Technol., 2014, 48, 9079–9085 CrossRef CAS PubMed.
- U. Bockelmann, H. H. Dorries, M. N. Ayuso-Gabella, M. S. de Marcay, V. Tandoi, C. Levantesi, C. Masciopinto, E. Van Houtte, U. Szewzyk, T. Wintgens and E. Grohmann, Appl. Environ. Microbiol., 2009, 75, 154–163 CrossRef PubMed.
- L. Rizzo, C. Manaia, C. Merlin, T. Schwartz, C. Dagot, M. C. Ploy, I. Michael and D. Fatta-Kassinos, Sci. Total Environ., 2013, 447, 345–360 CrossRef CAS PubMed.
- Y. L. Zhang, C. F. Marrs, C. Simon and C. W. Xi, Sci. Total Environ., 2009, 407, 3702–3706 CrossRef CAS PubMed.
- A. Schlüter, R. Szczepanowski, A. Pühler and E. M. Top, FEMS Microbiol. Rev., 2007, 31, 449–477 CrossRef PubMed.
- M. Munir, K. Wong and I. Xagoraraki, Water Res., 2011, 45, 681–693 CrossRef CAS PubMed.
- A. Liu, A. Fong, E. Becket, J. Yuan, C. Tamae, L. Medrano, M. Maiz, C. Wahba, C. Lee, K. Lee, K. P. Tran, H. Yang, R. M. Hoffman, A. Salih and J. H. Miller, Antimicrob. Agents Chemother., 2011, 55, 1204–1210 CrossRef CAS PubMed.
- G. McVicker, T. K. Prajsnar, A. Williams, N. L. Wagner, M. Boots, S. A. Renshaw and S. J. Foster, PLoS Pathog., 2014, 10, e1003959 Search PubMed.
- D. I. Andersson and D. Hughes, Nat. Rev. Microbiol., 2014, 12, 465–478 CrossRef CAS PubMed.
- E. Gullberg, L. M. Albrecht, C. Karlsson, L. Sandegren and D. I. Andersson, Ambio, 2014, 5, 19–23 Search PubMed.
- P. Y. Zhang, P. P. Xu, Z. J. Xia, J. Wang, J. Xiong and Y. Z. Li, FEMS Microbiol. Lett., 2013, 348, 149–156 CrossRef CAS PubMed.
- Z. J. Xia, J. Wang, W. Hu, H. Liu, X. Z. Gao, Z. H. Wu, P. Y. Zhang and Y. Z. Li, J. Ind. Microbiol. Biotechnol., 2008, 35, 1157–1163 CrossRef CAS PubMed.
- B. Song, G. R. Wang, N. B. Shoemaker and A. A. Salyers, J. Bacteriol., 2009, 191, 1078–1082 CrossRef CAS PubMed.
- M. Prudhomme, L. Attaiech, G. Sanchez, B. Martin and J. P. Claverys, Science, 2006, 313, 89–92 CrossRef CAS PubMed.
- C. Úbeda, E. Maiques, E. Knecht, Í. Lasa, R. P. Novick and J. R. Penadés, Mol. Microbiol., 2005, 56, 836–844 CrossRef PubMed.
- J. W. Beaber, B. Hochhut and M. K. Waldor, Nature, 2004, 427, 72–74 CrossRef CAS PubMed.
- C. Baker-Austin, M. S. Wright, R. Stepanauskas and J. V. McArthur, Trends Microbiol., 2006, 14, 176–182 CrossRef CAS PubMed.
- L. Sörme and R. Lagerkvist, Sci. Total Environ., 2002, 298, 131–145 CrossRef.
- S. K. Brar, M. Verma, R. D. Tyagi and R. Y. Surampalli, Waste Manage., 2010, 30, 504–520 CrossRef CAS PubMed.
- D. P. Karumathil, H.-B. Yin, A. Kollanoor-Johny and K. Venkitanarayanan, Int. J. Environ. Res. Public Health, 2014, 11, 1844–1854 CrossRef CAS PubMed.
- P. Shi, S. Jia, X. X. Zhang, T. Zhang, S. Cheng and A. Li, Water Res., 2013, 47, 111–120 CrossRef CAS PubMed.
- J. J. Huang, H.-Y. Hu, Y.-H. Wu, B. Wei and Y. Lu, Chemosphere, 2013, 90, 2247–2253 CrossRef CAS PubMed.
- A. Boehm, S. Steiner, F. Zaehringer, A. Casanova, F. Hamburger, D. Ritz, W. Keck, M. Ackermann, T. Schirmer and U. Jenal, Mol. Microbiol., 2009, 72, 1500–1516 CrossRef CAS PubMed.
- M. Blickwede, R. Goethe, C. Wolz, P. Valentin-Weigand and S. Schwarz, J. Antimicrob. Chemother., 2005, 56, 315–323 CrossRef CAS PubMed.
- D. Li, A. Renzoni, T. Estoppey, C. Bisognano, P. Francois, W. L. Kelley, D. P. Lew, J. Schrenzel and P. Vaudaux, Antimicrob. Agents Chemother., 2005, 49, 916–924 CrossRef CAS PubMed.
- L. R. Hoffman, D. A. D'Argenio, M. A. MacCoss, Z. Zhang, R. A. Jones and S. I. Miller, Nature, 2005, 436, 1171–1175 CrossRef CAS PubMed.
- M. Hausner and S. Wuertz, Appl. Environ. Microbiol., 1999, 65, 3710–3713 CAS.
- S. Molin and T. Tolker-Nielsen, Curr. Opin. Biotechnol., 2003, 14, 255–261 CrossRef CAS PubMed.
- T. Das, S. Sehar and M. Manefield, Environ. Microbiol. Rep., 2013, 5, 778–786 CrossRef CAS PubMed.
- Y. Li, P. C. Y. Lau, J. H. Lee, R. P. Ellen and D. G. Cvitkovitch, J. Bacteriol., 2001, 183, 897–908 CrossRef CAS PubMed.
- S. Hannan, D. Ready, A. S. Jasni, M. Rogers, J. Pratten and A. P. Roberts, FEMS Immunol. Med. Microbiol., 2010, 59, 345–349 CrossRef CAS PubMed.
- N. M. Vega and J. Gore, Curr. Opin. Microbiol., 2014, 21, 28–34 CrossRef CAS PubMed.
- A. Pruden, Environ. Sci. Technol., 2014, 48, 5–14 CrossRef CAS PubMed.
- N. J. Ashbolt, A. Amezquita, T. Backhaus, P. Borriello, K. K. Brandt, P. Collignon, A. Coors, R. Finley, W. H. Gaze, T. Heberer, J. R. Lawrence, D. G. J. Larsson, S. A. McEwen, J. J. Ryan, J. Schonfeld, P. Silley, J. R. Snape, C. Van den Eede and E. Topp, Environ. Health Perspect., 2013, 121, 993–1001 Search PubMed.
- E. M. H. Wellington, A. B. Boxall, P. Cross, E. J. Feil, W. H. Gaze, P. M. Hawkey, A. S. Johnson-Rollings, D. L. Jones, N. M. Lee, W. Otten, C. M. Thomas and A. P. Williams, Lancet Infect. Dis., 2013, 13, 155–165 CrossRef CAS PubMed.
- H. K. Allen, J. Donato, H. H. Wang, K. A. Cloud-Hansen, J. Davies and J. Handelsman, Nat. Rev. Microbiol., 2010, 8, 251–259 CrossRef CAS PubMed.
- K. Rosario, C. Nilsson, Y. W. Lim, Y. Ruan and M. Breitbart, Environ. Microbiol., 2009, 11, 2806–2820 CrossRef CAS PubMed.
- M. Muniesa, M. Colomer-lluch and J. Jofre, Future Microbiol., 2013, 8, 739–752 CrossRef CAS PubMed.
- J. M. Thomas, N. J. Ashbolt, N. Exposure, U. States, E. P. Agency, J. M. Thomas and N. J. Ashbolt, Environ. Sci. Technol., 2011, 45, 860–869 CrossRef CAS PubMed.
- US CDC, Antibiotic Resistance Threats in the United States, 2013, p. 114 Search PubMed.
- B. Blair, P. Sarkar, K. R. Bright, F. Marciano-Cabral and C. P. Gerba, Emerging Infect. Dis., 2008, 14, 1498–1499 CrossRef PubMed.
- T. A. Bartrand, J. J. Causey and J. L. Clancy, J. - Am. Water Works Assoc., 2014, 106, E418–E432 CrossRef.
- A. J. Martinez and G. S. Visvesvara, Brain Pathol., 1997, 7, 583–598 CrossRef CAS.
- M. C. Liang, Z. G. Ning and Y. K. Li, Environ. Earth Sci., 2015, 73, 4325–4338 CrossRef CAS.
- V. Thomas, J. Loret, M. Jousset and G. Greub, Environ. Microbiol., 2008, 10, 2728–2745 CrossRef CAS PubMed.
- H. C. Miller, J. Wylie, G. Dejean, A. H. Kaksonen, D. Sutton, K. Braun and G. J. Puzon, Environ. Sci. Technol., 2015, 49, 11125–11131 CrossRef CAS PubMed.
- J. M. Thomas and N. J. Ashbolt, Environ. Sci. Technol., 2011, 45, 860–869 CrossRef CAS PubMed.
- J. Lu, H. Y. Buse, V. Gomez-Alvarez, I. Struewing, J. Santo Domingo and N. J. Ashbolt, J. Appl. Microbiol., 2014, 117, 905–918 CrossRef CAS PubMed.
- M. T. García, S. Jones, C. Pelaz, R. D. Millar and Y. Abu Kwaik, Environ. Microbiol., 2007, 9, 1267–1277 CrossRef PubMed.
- P. K. Jjemba, W. Johnson, Z. Bukhari and M. W. LeChevallier, Pathogens, 2015, 4, 470–502 CrossRef PubMed.
- J. L. Griffin, Science, 1972, 178, 869–870 CAS.
- H. Y. Buse and N. J. Ashbolt, Lett. Appl. Microbiol., 2011, 53, 217–224 CrossRef CAS PubMed.
- M. A. Söderberg, J. Dao, S. R. Starkenburg and N. P. Cianciotto, Appl. Environ. Microbiol., 2008, 74, 5583–5588 CrossRef PubMed.
- B. C. Hitzfeld, S. J. Höger and D. R. Dietrich, Environ. Health Perspect., 2000, 108, 113–122 CrossRef CAS PubMed.
- G. Codd, S. Bell, K. Kaya, C. Ward, K. Beattie and J. Metcalf, Eur. J. Phycol., 1999, 34, 405–415 CrossRef.
-
B. Mueller, New York Times, http://www.nytimes.com/2015/10/02/nyregion/legionnaires-bacteria-regrew-in-bronx-cooling-towers-that-were-disinfected.html?_r=0, (accessed January 2016).
- B. E. Hatt, A. Deletic and T. D. Fletcher, J. Environ. Manage., 2006, 79, 102–113 CrossRef PubMed.
- Austrailian Government National Health and Medical Research Council, 2004.
-
L. R. Brown, Full Planet, Empty Plates: The New Geopolitics of Food Scarcity, 2012 Search PubMed.
-
E. Schroeder, G. Tchobanoglous, H. L. Leverenz and T. Asano, Natl. Water Resesearch Inst. White Pap., 2012, pp. 1–20 Search PubMed.
- S. Adapa, N. Bhullar and S. Desouza, J. Cleaner Prod., 2016 DOI:10.1016/j.jclepro.2016.02.083.
- D. Baumann, Appl. Geogr., 1983, 3, 79–84 CrossRef.
-
J. G. Lohman and Milliken L.C., U.S. Dep. Inter. Denver, 1985.
- S. Dolnicar, A. Hurliman and L. D. Nghiem, J. Environ. Manage., 2010, 91, 1288–1293 CrossRef PubMed.
- K. P. Tsagarakis, A. N. Menegaki, K. Siarapi and F. Zacharopoulou, J. Risk Res., 2013, 16, 133–144 CrossRef.
-
T. Asano, F. L. Burton, H. L. Leverenz, R. Tsuchihashi and G. Tchobanoglous, Water Reuse: Issues, Technologies, and Applications, McGraw-Hill, New York City, 2016 Search PubMed.
- R. E. Rosenberg Goldstein, S. A. Micallef, S. G. Gibbs, J. A. Davis, X. He, A. George, L. M. Kleinfelter, N. A. Schreiber, S. Mukherjee, A. Sapkota, S. W. Joseph and A. R. Sapkota, Environ. Health Perspect., 2012, 120, 1551–1558 CrossRef PubMed.
- M. W. LeChevallier and R. J. Seidler, Appl. Environ. Microbiol., 1980, 30, 739–742 Search PubMed.
- P. Rusin, J. Rose, C. Haas and C. Gerba, Rev. Environ. Contam. Toxicol., 1997, 152, 57–83 CAS.
- F. Xianmin, Z. Wenyu, W. Yuehua, Z. Donghai, J. Xiaoming and L. Shijie, Anat. Rec., 2015, 298, 1509–1517 CrossRef PubMed.
-
P. K. Jjemba, L. Weinrich, W. Cheng, E. Giraldo and M. W. LeChevallier, Guidance Document on the Microbiological Quality and Biostability of Reclaimed Water Following Storage and Distribution, 2010 Search PubMed.
-
L. Macmillian, New York Times Green Blog, http://green.blogs.nytimes.com/2012/10/10/do-not-publish-on-the-slopes-gliding-on-wastewater/?_r=0, (accessed January 2016).
- Q. Y. Wu, Y. Y. Li and H. Y. Hu, Huanjing Kexue Xuebao, 2013, 33, 844–849 CAS.
- T. W. Armstrong and C. N. Haas, Risk Anal., 2007, 27, 1581–1596 CrossRef CAS PubMed.
- T. P. Primm, C. A. Lucero and J. O. Falkinham, Clin. Microbiol. Rev., 2004, 17, 98–106 CrossRef PubMed.
- B. J. Williams, J. Dehnbostel and T. S. Blackwell, Respirology, 2010, 15, 1037–1056 CrossRef PubMed.
- Y. J. Lee, J. H. Kim, H. J. Sohn, J. Lee, S. Y. Jung, Y. J. Chwae, K. Kim, S. Park and H. J. Shin, Parasite Immunol., 2011, 33, 382–389 CrossRef CAS PubMed.
- H. Ryu, A. Alum and M. Abbaszadegan, Environ. Sci. Technol., 2005, 39, 8600–8605 CrossRef CAS PubMed.
- C. W. Knapp, S. M. McCluskey, B. K. Singh, C. D. Campbell, G. Hudson and D. W. Graham, PLoS One, 2011, 6, e27300 CAS.
- M. J. Mohammad Rusan, S. Hinnawi and L. Rousan, Desalination, 2007, 215, 143–152 CrossRef CAS.
- B. Kurenbach, D. Marjoshi, C. F. Amábile-cuevas, G. C. Ferguson, W. Godsoe, P. Gibson and J. A. Heinemann, mBio, 2015, 6, 1–9 CrossRef CAS PubMed.
- M. Salgot, E. Huertas, S. Weber, W. Dott and J. Hollender, Desalination, 2006, 187, 29–40 CrossRef CAS.
-
J. K. Edzwald, Water Quality & Treatment: A Handbook on Drinking Water, McGraw-Hill, New York City, 6th edn, 2011 Search PubMed.
- C. Volk, C. Renner, C. Robert and J. C. Joret, Environ. Technol., 1994, 15, 545–556 CrossRef CAS.
-
P. Servais, P. Laurent and D. Gatel, presented in part at Proc. of the Annual AWWA Water Qual. Technol. Conf., New Orleans, 1995 Search PubMed.
- P. Laurent, M. Prévost, J. Cigana, P. Niquette and P. Servais, Water Res., 1999, 33, 1387–1398 CrossRef CAS.
- P. Niquette, P. Servais and R. Savoir, Water Res., 2001, 35, 675–682 CrossRef CAS PubMed.
- C. J. Volk and M. W. LeChevallier, J. Am. Water Works Assoc., 2000, 92, 64–76 CAS.
- D. van der Kooij, A. Visser and W. A. M. Hijnen, J. Am. Water Works Assoc., 1982, 74, 540–545 CAS.
- M. W. LeChevallier, W. Schulz and R. G. Lee, Appl. Environ. Microbiol., 1991, 57, 857–862 CAS.
- M. Vital, H. P. Fuchslin, F. Hammes and T. Egli, Microbiology, 2007, 153, 1993–2001 CrossRef CAS PubMed.
- D. Gerrity, S. Gamage, J. C. Holady, D. B. Mawhinney, O. Quiñones, R. A. Trenholm and S. A. Snyder, Water Res., 2011, 45, 2155–2165 CrossRef CAS PubMed.
- Ç. Kalkan, K. Yapsakli, B. Mertoglu, D. Tufan and A. Saatci, Desalination, 2011, 265, 266–273 CrossRef.
- A. N. Pisarenko, B. D. Stanford, D. Yan, D. Gerrity and S. A. Snyder, Water Res., 2012, 46, 316–326 CrossRef CAS PubMed.
Footnote |
† These authors contributed equally to this work. |
|
This journal is © The Royal Society of Chemistry 2016 |