Development and field test of a mobile continuous flow system utilizing Chemcatcher for monitoring of rare earth elements in marine environments†
Received
12th May 2015
, Accepted 23rd September 2015
First published on 24th September 2015
Abstract
An important limiting factor that hampers accurate determination of the time-weighted average (TWA) concentrations of aqueous micropollutants by passive sampling is unsteady water turbulence, which can cause high variations of the uptake rates of target substances by the samplers during their field exposure. A mobile continuous flow system (MCFS) was developed for the improved utilization of the Chemcatcher passive sampler for time-integrated field monitoring of metals under controlled and reproducible hydrodynamic conditions. The mobile system is based on a specifically designed stirring system integrated in a flow-through cell. This design enables the adjustment of hydrodynamic conditions around the samplers as used in the laboratory calibration experiments. By using this system, the reliability of monitoring data can be improved. A prototype of this mobile continuous flow system was tested during a research cruise in May 2014 (HE422) to collect TWA concentrations for rare earth elements (REEs). The field test covered two areas in the German Bight of the North Sea. The Chemcatcher® results yield TWA concentration levels of REEs between 3.9 ng L−1 and 19.4 ng L−1 for the off-shore (low anthropogenic influence) and between 7.0 ng L−1 and 38.6 ng L−1 for the near-shore (high anthropogenic impact) areas, while the direct measurements of the ultra-trace levels of the REEs in the seawater spot samples were nearly impossible. The successful application of the developed MCFS demonstrated that monitoring with passive samplers on ships is possible with high accuracy under controlled hydrodynamic conditions even at the ultra-trace element concentration levels as typical for off-shore areas.
Water impact
Rare earth elements are emerging analytes in marine environments but appear at ultra-trace levels only. We designed a mobile continuous flow system for deploying passive samplers under reproducible hydrodynamic conditions in pumped seawater aboard a ship. Field testing of the system during a cruise in the North Sea yielded precise time-weighted average concentrations of the analytes even in off-shore areas.
|
1. Introduction
The application of passive samplers has gained a strong interest during recent years. Several passive sampler devices were described for various kinds of contaminant classes. Depending on the exposure time in the field and the sampler design, the mass of analyte which is accumulated in the receiving phase of a passive sampler reflects an equilibrium or a time-weighted average (TWA) concentration.1 Commonly used equilibrium passive samplers for the accumulation of metals are the SAFIR2 or the Gellyfish.3 Two passive samplers working in the kinetic uptake regime for the monitoring of metals are the diffusive gradients in thin films (DGT)4 and the Chemcatcher®-Metal.5 The DGT was developed in 1994 by Zhang and Davison.4 The sampler is based on a layer of Chelex-100 resin impregnated in a hydrogel. The resin layer is overlaid with a hydrogel, which acts as diffusion barrier, and a cellulose nitrate filter to prevent binding of fine particles on the hydrogel surface.4 The Chemcatcher®-Metal consists of a PTFE sampler body, which holds a 3M Empore chelating disk as the receiving phase overlaid by a cellulose acetate membrane as diffusion-limiting barrier.6 The mass transfer into a passive sampler is driven by the chemical potential gradient existing between the bulk water and the sampling phase. This transport process is influenced by the hydrodynamic conditions near the sampler surface which are critical for the determination of reliable TWA concentrations. The uncertainty in TWA concentrations is least when the field sampling conditions can be tuned comparably to those utilized in lab-based calibration studies for generating the sampling rates.7 However, this seems to be an impossible undertaking in many turbulent aqueous environments. In order to use a passive sampler in marine waters with high reproducibility and accuracy for routine monitoring, it is necessary to limit the influence of fluctuating hydrodynamic conditions during the sampling. Therefore, a mobile continuous flow system (MCFS) was developed, and is presented in this study. The system enables the control of the water flow around the exposed samplers and is, therefore, independent from often varying hydrodynamic conditions during the exposition process. Another advantage of the system is the possibility to use it while mobile on a research or shipping vessel to perform monitoring in off-shore regions, where it will be challenging to use conventional sampling methods or to deploy passive samplers. To show the benefit of the novel mobile continuous flow system, it was tested on a research vessel cruise during a 14-day trial to determine rare earth elements (REEs) using the Chemcatcher®-Metal sampler.
Due to their specific properties, the use of REEs offers a wide range of application fields such as in metallurgy and alloys, in electronics, as chemical catalysts or for glass polishing, ceramics and other purposes, as well as for an increasing number of new technologies in the “clean energy sector” (i.e., wind turbines and hybrid automobiles), etc.8 As such, the global production of REEs has strongly increased.9 The concentrations of REEs in the aquatic environment are not at the acute toxic levels, so the most likely mechanism for their biological adverse effects is their long-term bioaccumulation in aquatic organisms and enrichment through the food web.8,10 The effect of these contaminants may be small as they do not necessarily cause direct mortality but they may have negative population effects via their influence on reproduction processes and larval viability, etc.11,12
The water concentrations of REEs in the German Bight are expected to be in a low ng L−1 level. Due to this low concentration level and the high salt content in the water sample, environmental analysis of REEs in coastal waters is a challenging task. Conventionally, REEs in waters were detected by spot sampling followed by several preconcentration steps; for example, complexation with chelating agents before measurement.13,14 Passive sampling could be a solution to save time in sample pre-concentration and preparation and give a TWA concentration of the REEs, in contrast to the traditional spot sampling which presents only an instantaneous “snapshot” of the water quality for the sampling moment.15 To our knowledge, no work has been published on passive sampling of REEs in the German Bight or elsewhere at sea.
2. Materials and methods
2.1. Chemicals
A certified, custom made ICP-MS multi-element standard solution (ESI-37) was obtained from Inorganic Ventures (Christiansburg, USA). Scandium, yttrium and indium were obtained as single-element standards from Sigma-Aldrich (St. Louis, USA).
The Suprapur® 65% nitric acid (HNO3) used was purchased from Th. Geyer (Hamburg, Germany). All equipment was thoroughly acid cleaned prior to use. The Suprapur® HNO3 was further purified two times by sub-boiling distillation in a quartz still (AHF Analysentechnik, Tübingen, Germany) to further reduce any trace element contaminations. All the preparation and extraction steps of the Chemcatcher® were performed inside a class 100 clean bench, which was placed in a class 10
000 clean room to minimize blank levels and any contamination risks. The ultra-pure water used for cleaning and analyses was obtained from a Milli-Q ElementQ water system (Merck-Millipore, Darmstadt, Germany).
2.2. Description of the sampling area
The field test was performed in the German Bight during a cruise with the research vessel (RV) Heincke (a property of the Helmholtz Centre for Marine and Polar Research (AWI); cf.http://www.awi.de/en/expedition/ships/research-vessel-heincke.html) in May 2014 (HE422 cruise). Two areas with different anthropogenic impacts were selected. Area A is an off-shore area and less-impacted, while area B is near-shore and highly influenced by industrial activities. Fig. 1 shows a map of both sampling areas and the anthropogenic impact e.g. from installed wind farms (pink) and highly frequented ship traffic routes (blue lines).
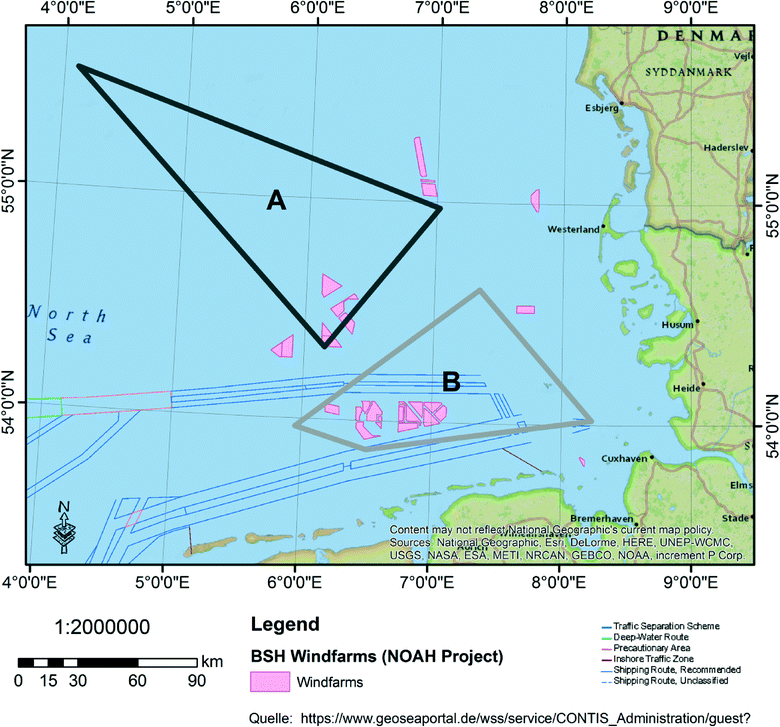 |
| Fig. 1 Map of the sampling area investigated during the ship campaign (HE422) in May 2014: sampling area A is off-shore coastal waters and sampling area B is near-shore coastal waters. The pink color represents existing wind farms and the blue lines indicate the main ship traffic routes (data: BSH – Federal Maritime and Hydrographic Agency, NOAH – North Sea Observation and Assessment of Habitat). | |
2.3. Development and characteristics of the mobile continuous flow system
The newly developed exposure system named the mobile continuous flow system (MCFS) (*patent pending) is presented in Fig. 2. The system is particularly designed for the sampling of trace metals in seawater, so only pure and precleaned polypropylene (PP), polytetrafluoroethylene (PTFE), perfluoroalkoxyalkanes (PFA) and titanium were used as materials. This helped to minimize the blank contributions from the system as well as to prevent corrosion. The main part the prototype is a flow-through cell containing two passive sampler holders and a stirring system (Fig. 3, top right). The flow-through cell is made of high purity polypropylene and consists of three different parts, which could be easily disassembled, e.g. for cleaning. The first versions of the passive sampler holders were constructed for the integration of Chemcatcher® and DGT bodies (Fig. 3, picture at the bottom right). Each sampler holder (made of titanium) is designed as a “click style” system, which can hold up to three Chemcatchers® and three DGTs. The sampler holders are integrated in the flow-through cell so that the sampler surfaces are subjected to the same water flow conditions. The decision to integrate both Chemcatchers® and DGTs in one prototype sampler holder was made due to the fact that these passive samplers can be equipped with different membranes (Chemcatcher®) or diffusion layer (DGT) and as well as receiving phases allowing for sampling of a multitude of possible analytes. Previous publications described the application of the DGT for metals,4,16 phosphorus17 or antibiotics.18 The Chemcatcher® was successfully used in the past for the accumulation of hydrophobic organic substances,19 metals6 or pesticides.20
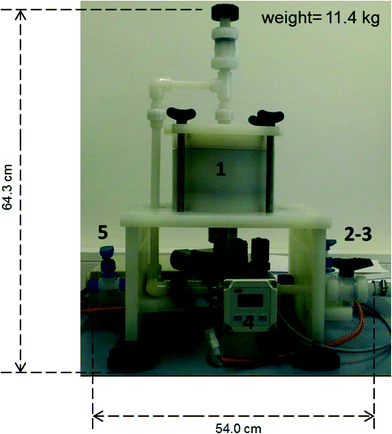 |
| Fig. 2 Photo of the mobile continuous flow system (MCFS). (1) Exposure box with a stirring system and a passive sampler holder; (2) inlet valve; (3) outlet valve; (4) water flow rate sensor; (5) outlet valve to allow water sampling and draining of the cell. | |
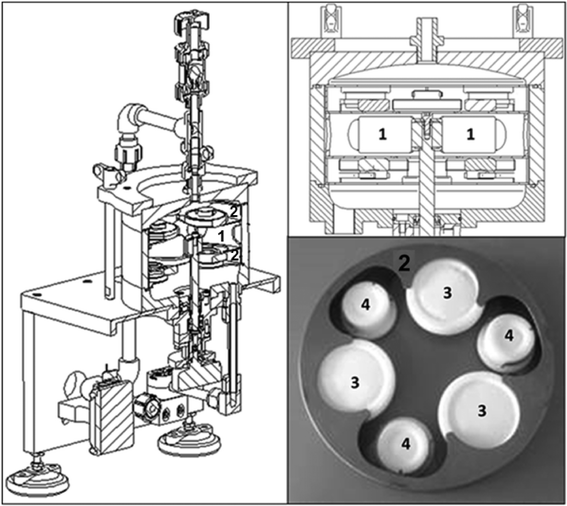 |
| Fig. 3 Left: Schematic profile of the MCFS. Right: Scheme and photo of the flow-through cell and the passive sampler holder (1) stirrer (diameter 112 mm); (2) sampler holder; (3) Chemcatcher®; (4) DGT. | |
The titanium stirrer is connected to a motor system. The mean diameter of the stirrer is 112 mm. The rotation speed (of the stirring system) is controlled via a computer program, which monitors and records the stability of the pre-set rotation speed to ensure constant hydrodynamic conditions independent from any water flow variations in the field.
Since the system is connected directly to the clean seawater inlet pump, the sampled water reflects the current trace element concentration level in the sampling area, which is currently crossed by the ship. Any external seawater pump system can be used to supply the water continuously into the flow-through cell via a pipe connection ending in an outlet valve after passing a flow-rate sensor. The flow-rate sensor (PeakTech FXL5000) was inserted to record the water flow rate at the outlet tube of the flow-through cell due to potential contamination by parts of the sensor.
2.4. MCFS installation on-board
The MCFS prototype was installed in a so-called wet laboratory on board the research ship RV Heincke. The system was connected to the clean seawater supply of the ship. The seawater is sucked from a depth of approximately 3 to 4 m using a metal-free polyethylene pump. All pipe connections inside the ship were made from polyvinyl chloride (PVC-U). Important oceanographic parameters such as salinity, temperature and pH of water flowing out of the MCFS were measured using a FerryBox system.21 These water quality data monitored during the passive sampler exposure time are given in Table 1.
Table 1 Average values of the physico-chemical water parameters based on the continuous measurement (1 measurement per minute) with a FerryBox in the German Bight in the North Sea
Parameter |
Sampling area A 03/05/2014, 3 a.m. – 09/05/2014, 9:40 a.m. (n = 9101) |
Sampling area B 09/05/2014, 10 a.m. – 14/05/2015, 8 a.m. (n = 5700) |
Salinity [PSU] |
34.4 ± 0.4 |
32.3 ± 1.4 |
Temperature [°C] |
14 ± 1 |
17 ± 1 |
pH |
8.2 ± 0.6 |
8.2 ± 0.1 |
A scheme of the whole experimental setup is presented in Fig. 4. Before the REE monitoring, the system was running for six hours without passive samplers to flush it with seawater. Six Chemcatchers® were exposed inside the MCFS and a stirring rate of 30 rpm was applied during the sampling at each area. In sampling area A, the samplers were exposed for 152 h and in area B for 118 h. Three samplers were prepared as procedural blanks for each sampling area and stored in demineralized water. 500 mL of seawater samples were collected semi-daily from a separate PFA outlet valve installed before the flow sensor of the MCFS (see Fig. 4).
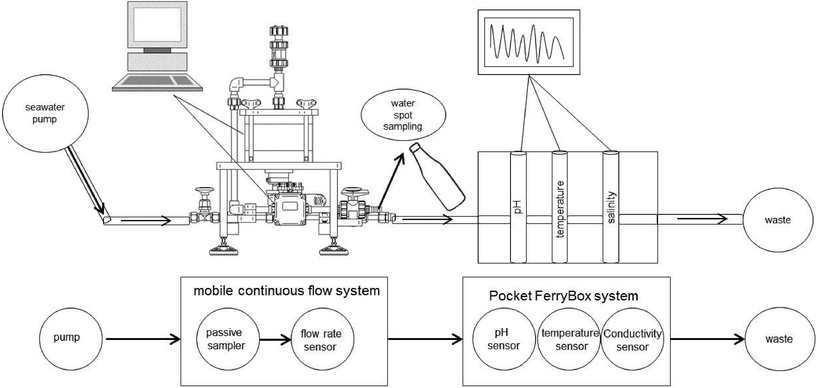 |
| Fig. 4 Schematic view indicating the integration of the mobile continuous flow system at the RV Heincke during the sampling campaign HE422 in May 2014. | |
2.5. Preparation and extraction of the Chemcatcher®-Metal
The Chemcatcher® body parts (thin version) were purchased from the University of Portsmouth, UK. 3M Empore™ Chelating Disks (47 mm in diameter) were purchased from Varian Inc. (Walton-on-Thames, UK). The cellulose acetate membrane material (40 μm in thickness, 0.45 μm in pore size) was obtained from Fisher Scientific (Loughborough, UK). The Chemcatcher® housing was cleaned by soaking it in 1 M HNO3 for one week followed by three rinsing steps with ultra-pure water. The Empore™ disks, used as the receiving phases, were conditioned with 20 mL of ultra-pure water and rinsed afterwards with 50 mL of 3 M HNO3, followed by three additional washing steps with ultra-pure water. To transform the Empore™ Chelating Disks to their active form, 100 mL of a 0.1 mol L−1 high purity ammonium acetate solution (pH = 5.3) was passed through each disk, followed by three washing steps with ultra-pure water. All steps were performed using a pre-cleaned 47 mm polycarbonate pressure filtration device (Sartorius, Göttingen, Germany). The cellulose acetate membranes were stored overnight in ultra-pure water before use. After the cleaning and conditioning steps, the Chemcatcher® was assembled inside a clean bench and stored in ultra-pure water at a 4 °C until use. After the exposure, the receiving phase was carefully removed from the Chemcatcher® housing, cleaned with ultra-pure water and finally extracted by using 30 mL of 3 M HNO3. As an internal standard, 50 μL of indium (1 ng μL−1) was added to the extract.
The TWA concentration was calculated using eqn (1):
| 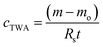 | (1) |
where
m is the mass of analyte accumulated by the receiving phase,
mo the mass in the blank (representing the possible background contamination during the sampler handling in the laboratory and field),
t the exposure time,
Rs the laboratory sampling rate and
cTWA the TWA concentration. The REE sampling rates used for the calculation of the TWA concentrations were determined in the laboratory by flow-through tank experiments in a titanium tank system at a water temperature of 13 °C and a stirrer speed of 30 rpm (comparable to the linear flow velocity of 0.5 m s
−1 at the sampler surface) as recently published by Petersen
et al. (2015).
22 Additionally, the sampling rates for Y (0.6 ± 0.2 mL h
−1) and Sc (1.9 ± 0.6 mL h
−1) were used (determined in a similar manner under the same conditions as above).
2.6. Preparation of the seawater samples
The water samples were filtered immediately after sampling using pre-cleaned Nucleopore® polycarbonate membrane filters (0.4 μm pore size, Whatman, Kent, UK) installed in a Sartorius pressure filtration unit. The filtrate was acidified with 500 μL of conc. HNO3 and stored in the dark at 4 °C. All sample handling was performed on board inside a class 100 clean bench to prevent any contaminations due to the ship environment. The measurement of the samples was performed using an Agilent 8800-ICP-MS-MS instrument two weeks after the sampling campaign. Each sample was measured in triplicate. The high matrix introduction system (HMI) of the ICP-MS-MS instrument was used to reduce the matrix input into the ICP. The sample aerosol was diluted with the HMI-mode on-line 10 times with argon gas.
2.7. Instrumental analysis
Analysis of the REEs was performed using an Agilent 8800-ICP-MS-MS (Agilent Technologies, Waldbronn, Germany), coupled to an ESI SC-4 DX Fast-autosampler (Elemental Scientific, Mainz, Germany). A Capricorn argon humidifier (Perkin Elmer, Rodgau, Germany) was inserted to prevent salt build up inside the sample introduction system. The instrument was optimized in a daily routine using a 10 ng mL−1 tuning solution, containing Li, Y, Ce and Tl, to maintain a consistent and reliable performance. The general instrumental settings for the measurements of the water samples and the passive samplers are summarized in Table 2.
Table 2 Instrumental settings of the Agilent 8800-ICP-MS-MS for the measurement of Chemcatcher® extracts and spot samples containing a high salt content
Parameter |
Chemcatcher® extracts |
Spot samples |
Sample introduction system |
— |
Argon humidifier |
Spray chamber |
Quartz Scott |
Quartz Scott |
Nebulizer |
Concentric MicroMist |
Concentric MicroMist |
Interface |
Ni sample cone |
Ni sample cone |
Ni skimmer |
Ni skimmer |
Lenses |
cx |
cx |
Plasma mode |
General purpose |
High matrix introduction mode |
Table S1 in the ESI† includes the LOD for all REEs, scandium and yttrium for seawater and the Chemcatcher®-Metal using an Agilent 8800-ICP-QQQ instrument. The LOD is based on the measurement of 10 Chemcatcher blanks (LOD Chemcatcher) and 10 filter blanks (LOD seawater). For each analyte, the limit of detection (LOD) was calculated as three times the standard derivation based on 10 replicates.
3. Results and discussion
3.1. Physical behavior of the MCFS during the utilization on-board the research vessel
The prototype of the MCFS showed a faultless operation during the 14-day ship campaign. The stirrer speed and the water flow were recorded continuously during the exposure of the passive samplers. The system worked even on rough seas. Changing of the passive samplers between the two sampling areas was easy due to the “click system”. No significant biofouling at the surface of the diffusion limiting membrane of the Chemcatchers was found during the whole exposure period. However, a salt crust was formed on the motor of the stirrer system at the underpart of the box. For further applications, it will be meaningful to construct a protection around the motor system to prevent salt precipitation and extend the service life of the motor. Furthermore, it will be much easier to integrate the pH, temperature and salinity sensors directly into the mobile continuous flow system instead of a connection with a FerryBox system. This modification will save space and time due to the fact that no extra instrument is necessary to measure the physico-chemical parameters. The successful testing of the MCFS opens a broad field of future applications for mobile passive sampling.
3.2. Comparison of the TWA concentrations determined with Chemcatcher® and the spot samples
For comparison, the results of the spot samples for the different areas have to be averaged. Such averaging can be critical, especially when some concentrations in the time series are under the limit of detection.23Table 3 shows the TWA concentrations of selected trace metals found in the Chemcatcher® extracts as well as the averaged results of the spot samples, which were taken during the Chemcatcher exposure. Most REEs could not be detected in the spot samples.
Table 3 Results of the field study using Chemcatcher®, integrated in the MCFS, for chosen trace metals in the German Bight. The results are presented as average ± standard derivation. The entry “n. d.” means “not detected” and in parentheses, the actual limit of detection is given
Element |
Sampling area Aa TWA concentration Chemcatcher® n = 6 [ng L−1] |
Sampling area Bb TWA concentration Chemcatcher® n = 6 [ng L−1] |
Sampling area Aa spot samples n = 12 [ng L−1] |
Sampling area Bb spot samples n = 10 [ng L−1] |
Off-shore seawater (close to no anthropogenic impact).
Near-shore seawater (industrial influenced).
|
Sc |
15.5 ± 5.6 |
33.1 ± 15.3 |
n. d. (6.0) |
n. d.(6.0) |
Y |
20.6 ± 3.9 |
29.5 ± 3.9 |
10.4 ± 1.2 |
13.8 ± 3.0 |
La |
10.4 ± 3.9 |
16.8 ± 3.9 |
n. d. (2.7) |
3.8 ± 1.9 |
Ce |
11.5 ± 4.3 |
19.7 ± 4.3 |
n. d. (3.2) |
5.5 ± 4.4 |
Pr |
5.2 ± 2.7 |
9.5 ± 2.7 |
n. d. (2.7) |
n. d. (2.7) |
Nd |
14.3 ± 6.4 |
24.3 ± 6.4 |
3.5 ± 2.3 |
5.7 ± 2.5 |
Sm |
7.5 ± 4.1 |
14.3 ± 4.1 |
n. d. (3.2) |
n. d. (3.2) |
Eu |
6.1 ± 3.5 |
10.8 ± 3.5 |
n. d. (2.7) |
n. d. (2.7) |
Gd |
10.0 ± 5.3 |
17.5 ± 5.3 |
n. d. (2.6) |
n. d. (2.6) |
Tb |
4.4 ± 2.9 |
7.9 ± 2.9 |
n. d. (2.5) |
n. d. (2.5) |
Dy |
10.1 ± 4.8 |
18.3 ± 4.8 |
n. d. (1.9) |
n. d. (1.9) |
Ho |
4.4 ± 2.6 |
8.1 ± 2.6 |
n. d. (2.4) |
n. d. (2.4) |
Er |
7.6 ± 3.8 |
14.3 ± 3.8 |
n. d. (2.9) |
n. d. (2.9) |
Tm |
4.4 ± 2.7 |
7.9 ± 2.7 |
n. d. (2.5) |
n. d. (2.5) |
Yb |
10.7 ± 5.1 |
18.3 ± 5.1 |
n. d. (2.5) |
n. d. (2.5) |
Lu |
4.0 ± 2.3 |
7.0 ± 2.3 |
n. d. (2.3) |
n. d. (2.3) |
In comparing spot sampling and passive sampling results, one should consider that the two sampling results represent different metal fractions in the water column. Passive samplers accumulate only the dissolved fraction of metals whereas spot sampling yields results which include also the fractions bound to particles and colloids, which are smaller than the pore size of the used filtration membrane. In general, the metal concentration of the spot samples is expected to be higher than the TWA concentrations obtained from passive sampling. This perception is difficult to prove when dealing with ultra-trace metal levels like the REEs in seawater. Direct measurement of seawater samples is challenging due to their high salt content and the resulting interferences on the REE masses. It was necessary to dilute the samples in this study by a factor of 10. The results of the spot samples, presented in Table 3, show that the concentration levels of the REEs are too low for a direct measurement of the filtrates. Application of preconcentration methods of the water sample before measurement can be a solution to detect such ultra-low concentration levels in spot samples.24–27 Regardless, pre-concentration is a time and labor-consuming work and is of questionable value for such spot samples which yield only “snapshot” information about the water quality. In contrast, the application of the Chemcatcher yields significant TWA concentrations for all REEs and differences between off-shore and near-shore sampling area (due to their in situ enrichment). The TWA concentrations of the REEs in area A range from 3.9 ng L−1 to 19.4 ng L−1 and in area B from 7.0 ng L−1 to 38.6 ng L−1. The highest concentrations are found for scandium and yttrium. Nearly all elements have approximately twice the concentration in the near-shore area B (industrially influenced area) when compared to the off-shore area A (a rarely anthropogenic impacted area). The comparison between the spot and passive sampling showed the high potential of the Chemcatcher as an alternative monitoring instrument, when the focus is on ultra-low concentrated analytes in coastal waters.
To our knowledge, the results presented here are the first measurements of REEs with a passive sampler system in the German Bight. The findings from area A can be considered as “background concentrations” of REEs and as relevant initial data for future monitoring programs to determine if due to the application of REEs in new “green” technologies (like wind farms), more REEs are released into the marine environment.
3.3. Reliability of the TWA concentrations based on Chemcatchers exposed in the MCFS
The standard deviations of the TWA concentrations listed in Table 3 are simply calculated from the six Chemcatcher replicates exposed, processed and analysed in parallel. These numbers show the precision of the results under stipulated conditions28 (here the passive sampling inside the MCFS) but neither represent the general uncertainty nor the trueness of results. It is in the nature of sampling in heterogeneous aqueous environments that no reference concentrations of micropollutants can be specified for checking the bias of measuring results. But it is possible at least to consider the uncertainty budget in a more detailed fashion. Under the assumption of the validity of the linear uptake model (i.e.eqn (1)), which is surely fulfilled with our trace-level accumulations in the chelating Empore disks of the Chemcatchers far below their adsorption capacity, one has to take two additional sources of uncertainty into account – the sampling rate and the exposure time. Relative standard deviations (of around 30%) of the Rs values useful for these calculations are determined in our recently reported laboratory experiments.16 The uncertainty in the exposure time can be set realistically to ±0.5 h and this yields relative standard deviations below 0.5% of the exposure times. By applying the law of error propagation, one can calculate the combined uncertainty of the TWA concentrations. These numbers and results for the investigated REEs are listed in Tables S2 and S3 in the ESI.† It can be stated that the combined uncertainties are only 10 to 25% higher than the standard deviations shown in Table 3. These results confirm the high level of precision that can be reached under well-defined sampling conditions with sophisticated analytical instrumentation and adequate Rs values.
Beyond the formal uncertainty calculations which were only briefly touched here, we want to emphasize again the importance of choosing the appropriate sampling rates. If we take, for example, the Rs values from ref. 16 which were determined for other water flow conditions, i.e. at 0.25 and 1.0 m s−1 (instead of 0.5 m s−1), the TWA concentrations from the present REE masses would be nearly twofold overestimated or up to four times underestimated, respectively.
4. Conclusions
A new mobile continuous flow system for the integration of Chemcatcher devices was developed. The main advantage of this system is the stirrer, which allows deployment of the passive samplers under controlled hydrodynamic conditions as simulated in the laboratory studies. This reduces the uncertainty of the TWA concentrations for the target analytes (here the REEs) considerably and can improve the reliability of monitoring data, especially in marine pollution research. The system is robust and easy to install and, thus, is applicable on research vessels. Future research studies will expand the field studies and testing of different passive sampler devices (like DGTs, cf.Fig. 3) to confirm the first application results. The MCFS will also be used for the monitoring of other elements or marine pollutants.
Acknowledgements
The authors acknowledge the financial support of the NOAH Project funded by the Federal Ministry of Education and Research, Germany. We are grateful to Volker Langhans, who constructed the mobile continuous flow box according to our expectations. We thank Jan Bödewadt and Jürgen Buhrz for the development of the software for the mobile continuous flow system. The support of Martina Gehrung, Henrike Thomas and Hendrik Rust is gratefully acknowledged for the helpful information about FerryBoxes. The authors would like to thank Dr. Wilhelm Petersen for supplying a PocketFerryBox system and the workshop of the Helmholtz-Centre Geesthacht for manufacturing the mobile continuous flow box.
References
-
G. A. Mills, G. R. Fones, K. Booij and R. Greenwood, in Chemical Marine Monitoring, ed. P. Quevauviller, P. Roose and G. Verreet, Wiley-VCH, Chichester, United Kingdom, 1st edn, ch. 13, 2011, pp. 397–431 Search PubMed.
- L. Li, F. Pala, J. Haskins, K. Sukola and G. T. Wallace, Environ. Sci. Technol., 2011, 45, 5660–5667 CrossRef CAS PubMed.
- Z. Dong, C. G. Lewis, R. M. Burgess and J. P. Shine, Environ. Toxicol. Chem., 2015, 34, 983–992 CrossRef CAS PubMed.
- H. Zhang and W. Davison, Anal. Chem., 1995, 67, 3391–3400 CrossRef CAS.
- R. Aguilar-Martínez, M. M. Gómez-Gómez, R. Greenwood, G. A. Mills, B. Vrana and M. A. Palacios-Corvillo, Talanta, 2009, 77, 1483–1489 CrossRef PubMed.
- L. B. Persson, G. M. Morrison, J.-U. Friemann, J. Kingston, G. Mills and R. Greenwood, J. Environ. Monit., 2001, 3, 639–645 RSC.
- J. Knutsson, P. Knutsson, S. Rauch, T. J. Pettersson and G. M. Morrison, Environ. Sci.: Processes Impacts, 2013, 15, 2233–2239 CAS.
-
P. L. Goering, in Elements and their Compounds in the Environment, ed. E. Merian, M. Anke and M. Ihnat, Wiley-VCH, Weinheim, Germany, 2nd edn, ch. 14, 2004, pp. 867–878 Search PubMed.
-
J. B. Hedrick, in Minerals yearbook-2000, ed. U. S. G. Survey, Washington DC, USA, 2000, pp. 132–133 Search PubMed.
- T. J. Haley, J. Pharm. Sci., 1965, 54, 663–670 CrossRef CAS PubMed.
- S. Hao, W. Xiaorong, H. Zhaozhe, W. Chonghua, W. Liansheng, D. Lemei, L. Zhong and C. Yijun, Chemosphere, 1996, 33, 1475–1483 CrossRef.
- S. Hao, W. Xiaorong, W. Liansheng, D. Lemei, L. Zhong and C. Yijun, Chemosphere, 1997, 34, 1753–1760 CrossRef.
- J. Cho, K.-W. Chung, M. S. Choi and H.-J. Kim, Talanta, 2012, 99, 369–374 CrossRef CAS PubMed.
- K. Hennebrüder, R. Wennrich, J. Mattusch, H.-J. Stärk and W. Engewald, Talanta, 2004, 63, 309–316 CrossRef PubMed.
- R. Aguilar-Martínez, M. M. Gómez-Gómez and M. A. Palacios-Corvillo, Int. J. Environ. Anal. Chem., 2011, 91, 1100–1116 CrossRef.
- Ø. A. Garmo, O. Røyset, E. Steinnes and T. P. Flaten, Anal. Chem., 2003, 75, 3573–3580 CrossRef PubMed.
- H. Zhang, W. Davison, R. Gadi and T. Kobayashi, Anal. Chim. Acta, 1998, 370, 29–38 CrossRef CAS.
- C.-E. Chen, H. Zhang and K. C. Jones, J. Environ. Monit., 2012, 14, 1523–1530 RSC.
- B. Vrana, G. A. Mills, M. Kotterman, P. Leonards, K. Booij and R. Greenwood, Environ. Pollut., 2007, 145, 895–904 CrossRef CAS PubMed.
- R. Gunold, R. B. Schäfer, A. Paschke, G. Schüürmann and M. Liess, Environ. Pollut., 2008, 155, 52–60 CrossRef CAS PubMed.
- W. Petersen, H. Wehde, H. Krasemann, F. Colijn and F. Schroeder, Estuarine, Coastal Shelf Sci., 2008, 77, 296–307 CrossRef.
- J. Petersen, D. Pröfrock, A. Paschke, J. A. C. Broekaert and A. Prange, Environ. Sci. Pollut. Res., 2015 DOI:10.1007/s11356-015-4823-x , in press.
- I. J. Allan, J. Knutsson, N. Guigues, G. A. Mills, A.-M. Fouillac and R. Greenwood, J. Environ. Monit., 2008, 10, 821–829 RSC.
- G. Bayon, D. Birot, C. Bollinger and J. A. Barrat, Geostand. Geoanal. Res., 2011, 35, 145–153 CrossRef CAS.
- L. Halicz, I. Gavrieli and E. Dorfman, J. Anal. At. Spectrom., 1996, 11, 811–814 RSC.
- M. B. Shabani and A. Masuda, Anal. Chem., 1991, 63, 2099–2105 CrossRef CAS.
- T. J. Shaw, T. Duncan and B. Schnetger, Anal. Chem., 2003, 75, 3396–3403 CrossRef CAS PubMed.
-
Eurachem/EUROLAB/CITAC/Nordtest/AMC Guide: Measurement uncertainty arising from sampling: a guide to methods and approaches, Eurachem (2007), ed. M. H. Ramsey and S. L. R. Ellison, ISBN 978 0 948926 26 6 Search PubMed.
Footnote |
† Electronic supplementary information (ESI) available. See DOI: 10.1039/c5ew00126a |
|
This journal is © The Royal Society of Chemistry 2016 |