Graphene–carbon nanotube aerogel as an ultra-light, compressible and recyclable highly efficient absorbent for oil and dyes†
Received
9th June 2015
, Accepted 12th October 2015
First published on 13th October 2015
Nano impact
Increasing worldwide attention has been paid to pollution coming from the oil industry especially the frequent occurrence of oil spill. This calls for environmentally friendly, renewable and effective absorbent materials. The current study explores the effects of carbon nanotubes (CNTs) on the structures, morphologies, specific surface areas and hydrophobic properties of 3D graphene aerogels (GAs). The obtained ultra-light graphene–CNT hybrid aerogels exhibited excellent adsorption capacity to oil and dyes as well as reusability. The present work suggests the promising widespread applications of such hybrid aerogels in practical water purification and oil remediation.
|
1 Introduction
With the rapid development of economy, increasing attention has been paid to severe ecological and environmental problems. Among them, pollution from the oil industry has been a serious threat to the survival of human beings. In recent years, the frequent occurrence of oil spill has caused immeasurable loss to our living environment and economic development.1 For instance, in May 2010, the Gulf of Mexico oil spill had caused about 2000 square miles of contaminated areas and billions of dollars of pecuniary loss.2 Recently, adsorption has been considered as one of the most efficient and cost-effective methods for oil remediation, but conventional absorbents such as various inorganic absorbents, natural organic fibres and synthetic organic polymers tend to suffer from secondary pollution, poor selectivity and low adsorption capacity.3 Therefore, environmentally friendly, renewable and effective absorbent materials are urgently needed to deal with oil spill accidents.
Two-dimensional (2D) graphene with one-atom thickness, which is a single layer of graphite, has attracted worldwide attention in recent years due to its excellent nature properties such as high specific surface area, ultra-low density and superhydrophobicity.4 Recent studies revealed that it could be applied as an excellent potential material to deal with oil contamination and adsorption problems.5 Nevertheless, the 2D graphene film has poor stability, low porosity and low collection efficiency, which encountered many obstacles to the adsorption of contaminants.6 Consequently, hierarchical 3D GA architectures fabricated through self-assembly processes have been proposed to overcome the limitations of 2D graphene.7 GAs can not only maintain the original properties of graphene, but also possess additional advantages such as high porosity and high surface area. The adsorption capacity (Qwt) of GAs can be up to several hundreds, which is much higher than that of current commercial materials such as sponges, active carbon, wool fiber and zeolites.8 In addition, other 3D porous structures including CNT sponges, graphene sponges, CNT–graphene aerogels, carbonaceous nanofiber aerogels and polydimethylsiloxane (PDMS) sponges also received great consideration.9 Among them, CNTs and graphene are especially attractive because of their high adsorption capacity, reusability and environmental friendliness.
Generally, both CNTs and graphene have good toughness and high strength,9d but neat graphene and CNT aerogels have weak elasticity.10 Recently, the combination of CNTs and graphene to prepare 3D composite aerogels for oil adsorption has aroused great attention. For example, Gao et al.11 reported a “sol-cryo” method for the synthesis of carbon aerogels with a density of 0.16 mg cm−3 and these aerogels exhibited excellent mechanical properties. Besides, the adsorption capacity to crude oil can reach as high as 290 g g−1. Nevertheless, this protocol needs to use virulent hydrazine vapor as a deoxidizer, which is harmful to our environment. To overcome this drawback, Losic's group has developed a green and one-step approach using ferrous ions as the green reducing agent to fabricate GCAs under hydrothermal conditions.12 The obtained GCAs with adsorption capacities of 21–35 g g−1, depending on the density of the adsorbed organics, were able to adsorb oils. Although these materials reveal good performance based on continuous vacuum-assisted adsorption experiments, the reduced iron nanoparticles on the graphene sheets increased the density of the aerogels. Thus, the adsorption capacity of these materials was greatly reduced. Moreover, the mechanical strength of these aerogels was indistinct in this work. Therefore, the quest for a green and facile route to synthesize ultra-light GCAs with high adsorption capacity and mechanical strength is still a tremendous challenge.
In this paper, we report a facile and green approach to synthesize GCAs through a one-step hydrothermal redox reaction. The obtained aerogels possess ultra-light densities ranging from 6.2–12.8 mg cm−3 depending on the original concentration of the GO and CNT solution. The effects of the amount of CNTs on the structures, morphologies, specific surface areas, hydrophobic properties, adsorption capacity as well as mechanical properties of the GAs were investigated systematically. The incorporation of CNTs into the GAs can not only enhance their adsorption capacity but also improve their mechanical properties. Therefore, the obtained GCAs with optimized GO/CNT ratios exhibited excellent reusability and mechanical strength on the basis of absorption–distillation and absorption–combustion experiments, which are very important for practical water purification and oil remediation applications.
2 Experimental
2.1 Materials
Graphite powder (average lateral size of 500 μm) was purchased from Qingdao Jin Ri Lai Graphite Co., Ltd. Ethylenediamine (EDA), H2SO4 (98%), KMnO4, P2O5, H2O2 (30%), K2S2O8 and ethanol were purchased from Chengdu Kelong Chemical Co., Ltd. CNTs with a diameter of 40–60 nm were obtained from Shenzhen Nanotech Port Co., Ltd. High-purity milli-Q water was used in all experiments.
2.2 Preparation of GO
GO was prepared through oxidation of natural graphite according to a previously reported method.13 Firstly, natural graphite flakes (5 g) were added gradually to a 1
:
3 mixture of fuming nitric acid and sulfuric acid (200 mL). The mixture was magnetically stirred at room temperature for 24 h and then 1 L of water was added slowly to the mixture to collect the solids after filtration. The solid products were washed with water and dried at 60 °C. Then, the dried powders were transferred into an oven at 1000 °C for ca. 10 s. After that, 5 g of the powders, 300 mL of sulfuric acid, 4.2 g of K2S2O8 and 6.2 g of P2O5 were successively placed into a flask and the mixture was kept at 80 °C for 5 h. After cooling to room temperature, 2 L of water was poured slowly into the mixture, which was then filtered and washed with water using a 0.22 μm pore polycarbonate membrane. The obtained solids were dried in air at room temperature. After that, the solids were added to 200 mL of concentrated H2SO4 at 0 °C, then 15 g of KMnO4 was added slowly (about 1 h) under continuous stirring conditions. Then, the mixture was heated to 35 °C and stirred for 2 h. The mixture was then diluted with 2 L of water, followed by the addition of 10 mL of H2O2. The mixture was stored for 2 days and then the upper clarified liquid was removed. The precipitates were washed with water and 1 M HCl. Finally, the washed solution was calibrated (2.5 mg ml−1) for further experiments.
2.3 Preparation of GA and GCA
In a typical procedure, a certain amount of CNTs (75 mg) and 0.75 mg of polyvinylpyrrolidone (PVP) were added to 30 mL of ethanol and the resulting mixture was sonicated for 5 h to form a dispersion (2.5 mg ml−1).14 The GO and CNT solution with different mass ratios including 1
:
0, 10
:
1, 7
:
1, 3
:
1 and 5
:
3 and a total volume of 40 mL was mixed and stirred for 1 h to form a uniform GO–CNT dispersion. After that, 160 μL of EDA was added and this dispersion was transferred into a Teflon-lined stainless steel autoclave and heated at 120 °C for 12 h to prepare a graphene–CNT hybrid hydrogel. The resulting hydrogel was washed several times with high-purity milli-Q water and freeze-dried (−80 °C pre-cooling) for 48 h to obtain the GA and GCA.
2.4 Characterization
Powder X-ray diffraction (PXRD) was performed by using a PANalytical X'Pert diffractometer operated at 40 kV and 40 mA using Cu Kα radiation. The Raman spectra of the samples were analyzed on a micro-Raman 2000 system. Scanning electron microscopy (SEM) was performed on a Hitachi S-4800 microscope. Transmission electron microscopy (TEM) was carried out on a FEI Tecnai G2 20 microscope operated at 200 kV. Fourier transform infrared (FT-IR) spectra were recorded on a Nicolet 6700 spectrometer. The contact angle was tested by using a JY-82C video contact angle tester. The specific surface area (SSA) was determined through the MB adsorption method by UV-vis spectroscopy (Shimadzu UV-2600).15 The SSA of the aerogels was calculated using the following equation:
where NA is Avogadro's number (6.02 × 1023 mol−1), AMB is the covered area per MB molecule (typically assumed to be 1.35 nm2), C0 and Ce are the initial and equilibrium concentrations of MB, respectively, V is the volume of MB solution, MMB is the relative molecular mass of MB, and ms is the mass of the sample.
2.5 Adsorption capacity measurements
For the oil–water adsorption tests, firstly, toluene was stained with Sudan red and then 2 mL of the stained toluene was added to water. 5.8 mg of the prepared aerogel was placed into this solution. The adsorbents were removed after the oil or organics were completely adsorbed. To investigate the adsorption performance of the GCAs, oil (lube), polar organics (n-hexane, ethanol and toluene) and non-polar organics (phenixin) were selected for the measurements. The weight of the GCA adsorbents was recorded before immersing in oil. The adsorbents were weighed again after about 5 min of immersion. The adsorption capacity (Qwt) is the ratio of the final weight (m1) after full adsorption to the initial weight (m0) of the GCA and can be calculated as follows:
To test the adsorption performance over dyes, 17 mg of the GCA was suspended in 50 mL of MB or methyl orange (MO) solution (10 mg L−1) with slight magnetic stirring to maintain the shape of the aerogels. 2 mL of the suspension was taken every 1 h and the concentration of the dyes was determined by using a UV-5100 (Anhui Wanyi) spectrometer.
2.6 Mechanical strength and cyclic adsorption experiment
The mechanical strength test was carried out through the following steps: the obtained GCA was compressed to about 80% of its own height; after dislodging the compression, the prepared GCAs returned to their original shape quickly. The same process was repeated many times. Two kinds of adsorption cycle experiments were performed. The absorption–distillation and absorption–combustion experiments were carried out to evaluate the recycling performance.7b For the absorption–combustion experiment, the aerogel was immersed in the organic solvents for about 5 min and then the aerogel was ignited directly in the crucible until combustion of n-hexane was completed. This process was repeated 10 times. For the adsorption–squeezing experiment, n-hexane was dropped onto the surface of the aerogel until the adsorbent reached adsorption saturation and then the aerogel was squeezed to half of its height. The same process was repeated 10 times.
3 Results and discussion
3.1 Characterization of GA and GCA
The PXRD patterns of the GO, GA, CNTs and GCA with the GO/CNT mass ratio of 3
:
1 are shown in Fig. 1a. A strong diffraction peak at 2θ = 26.1° (d-spacing = 3.4 Å) was observed for CNTs, whereas GO exhibited a diffraction peak at 2θ = 9.9° with the corresponding interlayer spacing of 8.9 Å. In addition, a weak peak at 2θ = 20.2° was also observed, indicating that oxygen-containing groups have already been introduced during the oxidation process.12 After the reduction process, the peak at 2θ = 9.9° of GA disappeared. Meanwhile, a new broadened diffraction peak at 24.7° (d-spacing = 3.7 Å) was observed for GA, which is much lower than that for GO (d-spacing = 8.9 Å) but slightly larger than that for natural graphite (3.4 Å), confirming the development of graphitic structures.16 Moreover, these results revealed the existence of π–π stacking between the graphene sheets in GA, which is composed of inhomogeneously stacked graphene sheets.7b In addition, the diffraction patterns of GCA contained the diffraction peaks from both GA and CNTs, pointing out the successful incorporation of CNTs into the GA. The Raman spectra of GO, CNTs, GA and GCA (Fig. 1b) display two obvious characteristic peaks at 1335 cm−1 and 1590 cm−1 corresponding to D and G bands, respectively, which are due to the defects and disorders in GO. In addition, a weak band at ca. 2700 cm−1 was observed for all the studied samples. This band could be attributed to two phonon lattice vibrations in the graphitic structure.15a The intensity ratio of the D and G bands (ID/IG) of both GA (1.10) and GCA (1.09) is significantly enhanced compared to that of the GO precursor (1.02), indicating the improvement in the disordered graphene sheets.16 Moreover, the ID/IG of GCA (1.09) is larger than that of the CNTs (1.06) but smaller than that of the GA (1.10), which further demonstrated that the prepared GCA contained both GA and CNTs,17 matching well with the results from the PXRD measurements (Fig. 1a).
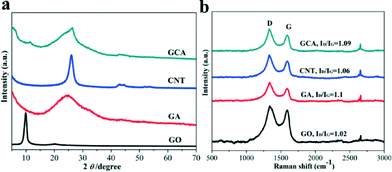 |
| Fig. 1 (a) PXRD patterns and (b) Raman spectra of GO, GA, CNTs and GCA. | |
To understand the effects of different amounts of CNTs on the morphologies of the GCAs, the samples with GO/CNT mass ratios of 1
:
0, 7
:
1, 3
:
1 and 5
:
3 were selected for further investigations. When the GO/CNT ratio was less than 5
:
3, it was difficult to form a hydrogel. The macroscopic structures of these aerogels (Fig. 2a–d) reveal a typical 3D network. The surface of GA was smooth and apparent cracks were observed (Fig. 2a). In the presence of CNTs, no cracks and exfoliation were observed on the surface. Besides, with increasing amounts of CNTs, the aerogels became denser (Fig. 2b–d). Meanwhile, the same phenomenon was observed in the SEM images (Fig. 2e–h). For pristine GA, most of the graphene sheets stacked severely and some large cracks among the stacked layers were observed as well (Fig. 2e). In contrast, the aerogels containing CNTs did not exhibit a serious accumulation phenomenon. Upon increasing the amount of CNTs, the graphene sheets became more discrete. Especially, porous structures with a size of several tens of micrometers appeared and the pore walls consisted of thin layers of a network with cross-linked graphene sheets and CNTs (Fig. 2f–h). Nevertheless, when the GO/CNT ratio reached 5
:
3, the graphene sheets and CNTs suffered from different degrees of agglomeration, leading to the destruction of the porous structures. Generally, the hydrothermal reduction process could promote the strong π–π interaction between the graphene sheets due to restoration of the conjugated carbon net. However, in the freeze-drying process, some graphene sheets could be separated by the growth force of the ice crystals, resulting in some obvious cracks in the aerogels. Therefore, the freeze-drying process has a remarkable impact on the morphology and structure of the final aerogels.18 For the hybrid aerogels, the CNTs played an important role as the skeleton and prevented fragmentation under the same freezing conditions. According to the high-magnification SEM image (Fig. S1†), it was observed that the CNTs in the aerogels play a role as the skeleton to support the graphene sheets. Furthermore, four kinds of existing forms between the CNTs and graphene sheets were found (Fig. S2†). Most of the CNTs are covered on the graphene wall to form a net framework (Fig. S2a†) and some of the CNTs act like sewing thread to connect two graphene sheets (Fig. S2b†). Apart from these, some CNTs are sandwiched in the middle of the graphene sheets (Fig. S2c†) and we also observed that some CNTs are wrapped by the graphene sheets (Fig. S2d†). Consequently, the multiple existing forms between the graphene sheets and CNTs could increase the surface roughness of the sheets, which is beneficial for the formation of a cross-linked structure between the layers.
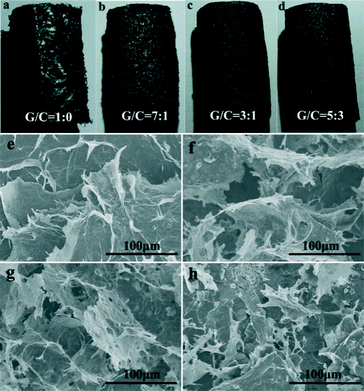 |
| Fig. 2 Photographs of the aerogels with GO/CNT mass ratios of (a) 1 : 0, (b) 7 : 1, (c) 3 : 1 and (d) 5 : 3; the corresponding SEM images of the aerogels with ratios of (e) 1 : 0, (f) 7 : 1, (g) 3 : 1 and (h) 5 : 3. | |
The TEM image of the GCA with a GO/CNT ratio of 3
:
1 (Fig. S3†) reveals the dense and homogeneous distribution of CNTs within the graphene sheets. The higher-magnification TEM image (Fig. 3a) further confirms that the CNTs with lengths ranging from several hundreds of nanometres to ten micrometers are tightly adhered on the graphene substrates and are quite flexible. The average diameter of the CNTs is ca. 50 nm. It is worth noting that the CNTs are sandwiched between the graphene sheets as observed from the edge of the graphene sheets (marked by a circle in Fig. 3a), which is consistent with the observations from the SEM image (Fig. S2c†). The high-resolution TEM (HRTEM) image (Fig. 3b) reveals that the obtained GCA has a well-graphitized structure. Three diffraction rings are observed in the selected area electron diffraction (SAED) pattern (inset in Fig. 3b). The corresponding d-spacings of these rings are 3.38 Å, 2.08 Å and 1.22 Å, respectively. All these results confirmed the crystalline nature of the prepared GCA.
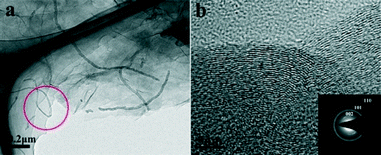 |
| Fig. 3 (a) TEM and (b) HRTEM images of GCA (inset is the corresponding SAED pattern). | |
It is well known that CNTs have excellent hydrophobic properties. Hence, the incorporation of CNTs into the GA could improve the surface hydrophobicity of the GA.19 As expected, upon increasing the amount of CNTs, the angle of contact with water increased from 40° to 114° (Fig. 4a–d). Fig. S4† shows the FT-IR spectra of GO, GA, CNTs and GCA. The graphene synthesized through the reduction of GO by EDA contained hydrophilic functional groups, such as O–H at 3430 cm−1, C–OH at 1389 cm−1, C
O at 1640 cm−1 attributed to stretching of the carbonyl (COOH) group and C–N at 1467 cm−1, which enhanced the interaction between graphene and water. Although the bands of COOH were greatly reduced after reduction from GO to GA, the contact angle of GA is only 40° because newly formed hydrophilic functional groups such as C–N were introduced during the reduction reaction process. The addition of superhydrophobic CNTs can not only increase the roughness of the GCA surface (Fig. 2), but also reduce the proportion of GO, leading to a significant improvement in the hydrophobicity of the GA. In addition, the SSAs of the GCAs with GO/CNT mass ratios of 1
:
0, 7
:
1, 3
:
1 and 5
:
3 determined by the MB adsorption method were 84.6 m2 g−1, 264.5 m2 g−1, 287.1 m2 g−1 and 127.0 m2 g−1, respectively (Fig. 4e). Obviously, the incorporation of CNTs into GA can also enhance the SSA, which can reach as high as more than 3-fold. Nevertheless, once the ratio of GO/CNT becomes higher than 3
:
1, the SSA of GCA decreases, which is in line with the macroscopic structures and SEM observations (Fig. 2).
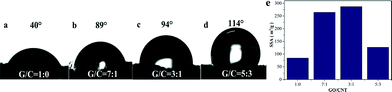 |
| Fig. 4 Water contact angle measurements of (a) GA, (b) GCA with the GO/CNT ratio of 7 : 1, (c) GCA with the GO/CNT ratio of 3 : 1 and (d) GCA with the GO/CNT ratio of 5 : 3; (e) the SSAs of these aerogels. | |
3.2 Adsorption capacity of GA and GCA
To investigate the selective adsorption properties of the prepared GCAs, oil–water separation experiments using toluene as the target adsorbate were performed. It was observed that toluene was immediately adsorbed. The completely selective adsorption process only took 90 s to accomplish (Fig. 5a). Moreover, adsorption experiments using pure oil in the absence of water were carried out to evaluate the effects of CNTs on the Qwt of GA (Fig. 5b). The Qwt of all the aerogels after the incorporation of CNTs increased remarkably compared to that of the pristine GA regardless of the density of the adsorbates such as n-hexane (0.692 g cm−3), toluene (0.866 g cm−3), lube (0.910 g cm−3) and phenixin (1.595 g cm−3). The Qwt of GA to phenixin and lube can reach ca. 200 and 140 times of its own weight, which are 5–15 times higher than that of conventional adsorbents such as sponges, active carbon, wool fiber and zeolites.8 The Qwt of the obtained GCA is not only further enhanced in comparison with that of GA but is also 3–8 times higher than those of the previously reported graphene–CNT aerogels.12 Among all the aerogels, the GCAs with GO/CNT mass ratios of 7
:
1 and 3
:
1 exhibit the best adsorption performance and the Qwt to lube and phenixin over these aerogels can reach as high as 190 and 270 g g−1, respectively, which is in line with the SEM observations and SSA measurements (Fig. 2 and 4). Consequently, the SSA is crucial for Qwt instead of the contact angle. Aside from hydrophobic organics, the obtained aerogels can even adsorb hydrophilic dyes.20Fig. 5c and d reveal the adsorption curves of two kinds of dyes (MB and MO) for the prepared aerogels. In line with the Qwt measurements, the GCAs with GO/CNT mass ratios of 7
:
1 and 3
:
1 also exhibited enhanced adsorption of MO and MB compared to other aerogels (Fig. 5c and d). Generally, the incorporation of CNTs into GA could promote the formation of microporous structures and thus enhance their SSAs. Nevertheless, when the GO/CNT mass ratio reaches 5
:
3, the CNTs aggregate severely and the graphene sheets are not sufficient to carry such a large amount of CNTs. Hence, the irregular distribution of CNTs and graphene sheets could result in the destruction of porous structures as well as a decrease in SSA. Such porous structures are crucial for the adsorption performance of the materials, because once the oils and organic solvents come into contact with the hydrophobic aerogels, they will penetrate into the aerogels and diffuse in the micropores of the aerogels, making the pore structure more conducive to spreading of the oil inside the aerogels. Therefore, the uniform pore can not only be a diffusion channel, but also wrap the oil inside the aerogels tightly.15a However, the order of the SSA was not entirely consistent with the adsorption capacity. For example, the SSA of the GCA with a ratio of 7
:
1 was slightly higher than that of the GCA with a ratio of 3
:
1, but the Qwt to lube and toluene were even lower. The same phenomenon also occurred in the GCAs with ratios of 1
:
0 and 5
:
3. This is probably because the adsorption capacity of the aerogels is not only determined by the SSA but is also related to the pore size of the aerogels and the properties of the target adsorbates, such as viscosity and surface tension.12,15a
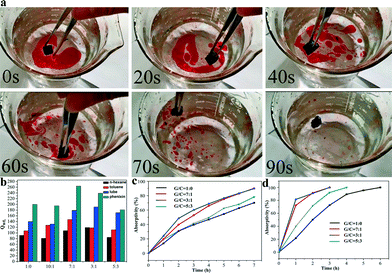 |
| Fig. 5 (a) The oil–water separation process of toluene (stained with Sudan red) from water by the GCA with the mass ratio of 3 : 1; (b) the Qwt of the GCAs with different GO/CNT ratios (1 : 0, 10 : 1, 7 : 1, 3 : 1 and 5 : 3). The black, red, blue and green rectangular frames represent n-hexane, toluene, lube and phenixin, respectively; (c and d) adsorption of MB and MO to the GCAs with different GO/CNT ratios (1 : 0, 10 : 1, 7 : 1, 3 : 1 and 5 : 3). | |
3.3 Reusability and cycling experiments of GA and GCA
The mechanical properties of the aerogels are very important for practical water purification and oil remediation applications. In order to investigate the mechanical properties of the obtained aerogels, the GCAs with GO/CNT mass ratios of 1
:
0 and 3
:
1 were selected for the mechanical strength measurements. The results are shown in Movie S1.† The GCA without CNTs exhibited poor resilience and the rebound process was accompanied by a hysteresis effect. However, the GCA with the GO/CNT ratio of 3
:
1 can fleetly rebound in the compression process. This is because the CNTs covered on the graphene sheets could enhance the tenacity of the aerogels. The GCA with CNTs possesses exceptional elastic properties as proven by the compression and rebound experiments, which is in favor of the recyclable performance of the aerogels. The treatment for oil leakage not only requires a high adsorption efficiency of oil–water separation materials but also needs suitable post-processing. Absorption–combustion has been considered as a direct and effective method to refresh oil-absorbing materials.7b Compared to traditional adsorptive materials such as polyester sponges and cotton, graphene has a very high melting point (over 3000 °C), which makes graphene aerogels potential materials for the absorption–combustion process. The sequential images (Fig. 6a–d and Movie S2†) show the absorption–combustion process for n-hexane. Remarkably, after combustion, the aerogels could still maintain their 3D structure. In order to investigate the role of CNTs in the aerogels, we also compared the cycling adsorption properties of GCA (GO/CNT = 3
:
1) and GA. According to the absorption–combustion cycling experiments (Fig. 6e and f), the GA was slightly fragile in comparison with the GCA after several times of combustion and the Qwt decreased by about 15% after the first cycle, whereas the GCA was extremely stable and almost no decrease in Qwt was observed even after 10 cycles. In addition, we further performed an absorption–combustion experiment using a highly viscous liquid (lube) with a viscosity of 100 mm2 s−1 (cf. Fig. S5†). Due to the high viscosity of the lube, the adsorbed liquid could not glidingly flow out from the aerogel and destroyed the aerogel to some extent. The results showed that the adsorption capacity decreased after 10 times of combustion. The adsorption capacity of the aerogel with a ratio of 3
:
1 decreased by about 33% and that with a ratio of 1
:
0 decreased by about 38%. Nevertheless, the GCA still exhibits outstanding reusability performance even after adsorbing such a highly viscous organic liquid (Fig. S5†).
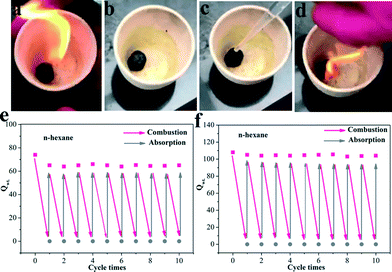 |
| Fig. 6 (a–d) Images of the absorption–combustion process; recyclability of the (e) GA and (f) GCA (GO/CNT = 3 : 1) for the adsorption of n-hexane under absorption–combustion cycles. | |
Nevertheless, the combustion of oils still causes environmental pollution and resource wastage. On the basis of the excellent elastic properties (Movie S1†), it is expected that the obtained aerogels can collect the oils from the water like a sponge. For this purpose, an adsorption–squeezing experiment was performed.7b The images (Fig. 7a and b and Movie S3†) demonstrated the cycling performance of our materials. Firstly, n-hexane was dropped onto the surface of the aerogels until the adsorbent reached adsorption saturation and then the aerogels were squeezed to half of their height to release the organics. Finally, when the aerogels quickly returned to their original height, n-hexane was re-dropped on the surface of the aerogels. The mechanical strength of the GCAs is good enough and thus almost no reduction of Qwt was observed after ten cycles of squeezing (Fig. 7f), while the GA displayed ca. a 13% decrease in Qwt after the cycling measurements (Fig. 7e). All in all, our prepared GCAs possess excellent mechanical strength, whether in the air or filled with organics. Hence, the GCAs exhibited a preferable resistance to combustion and compression compared with the GA.
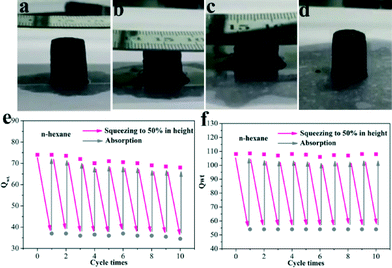 |
| Fig. 7 (a–d) Images of the adsorption–squeezing process; recyclability of the (e) GA and (f) GCA (GO/CNT = 3 : 1) for the absorption of n-hexane under adsorption–squeezing cycles. | |
4 Conclusions
In summary, ultra-light (6.2–12.8 mg cm−3) graphene–CNT aerogels were successfully prepared by a facile hydrothermal method using EDA as the reducing agent. The incorporation of CNTs into GA has significant influence on the structures, morphologies, SSAs, hydrophobic properties, adsorption capacity and mechanical properties of the GA. The multiple existing forms of CNTs in graphene sheets could increase the surface roughness of the sheets, thus enhancing the SSA from 84.6 to 287.1 m2 g−1. Therefore, the GCAs exhibited improved adsorption capacity to oil, organic solvents and dyes compared to the GA. When the GO/CNT mass ratios were 7
:
1 and 3
:
1, adsorption capacities 100–270 times of their own weight can be achieved depending on the density of the adsorbed organics. Importantly, the obtained GCAs exhibited excellent reusability and mechanical strength based on the absorption–distillation and absorption–combustion measurements. The GCAs can maintain their macroscopic shape and no decrease in adsorption capacity was observed even after 10 cycling experiments, which is very attractive for practical water purification and oil remediation.
Acknowledgements
Financial support from the Sichuan Youth Science and Technology Foundation (2013JQ0034), the National Natural Science Foundation of China (11405256, U1532120), the Innovative Research Team of Sichuan Provincial Education Department and SWPU (2012XJZT002), and the Open Foundation for the Key Laboratory of Oil and Gas Materials (x151514kcl30) is gratefully acknowledged.
Notes and references
-
(a) J. W. Short, S. D. Rice, R. A. Heintz, M. G. Carls and A. Moles, Energy Sources, 2003, 25, 509 CrossRef CAS;
(b) B. Dubansky, A. Whitehead, J. T. Miller, C. D. Rice and F. Galvez, Environ. Sci. Technol., 2013, 47, 5074 CrossRef CAS PubMed.
- M. Schrope, Nature, 2011, 472, 152 CrossRef CAS PubMed.
-
(a) M. O. Adebajo, R. L. Frost, J. T. Kloprogge, O. Carmody and S. Kokot, J. Porous Mater., 2003, 10, 159 CrossRef CAS;
(b) G. Deschamps, H. Caruel, M.-E. Borredon, C. Bonnin and C. Vignoles, Environ. Sci. Technol., 2003, 37, 1013 CrossRef CAS PubMed;
(c) H. B. Sonmez and F. Wudl, Macromolecules, 2005, 38, 1623 CrossRef CAS.
- A. K. Geim, Science, 2009, 324, 1530 CrossRef CAS PubMed.
- Y. X. Xu, Q. Wu, Y. Q. Sun, H. Bai and G. Q. Shi, ACS Nano, 2010, 4, 7358 CrossRef CAS PubMed.
- M. Toyoda and M. Inagaki, Spill Sci. Technol. Bull., 2007, 8, 467 CrossRef.
-
(a) M. A. Worsley, P. J. Pauzauskie, T. Y. Olson, J. Biener, J. H. Satcher and T. F. Baumann Jr., J. Am. Chem. Soc., 2010, 132, 14067 CrossRef CAS PubMed;
(b) J. H. Li, J. Y. Li, H. Meng, S. Y. Xie, B. W. Zhang, L. F. Li, H. J. Ma, J. Y. Zhang and M. Yu, J. Mater. Chem. A, 2014, 2, 2934 RSC;
(c) H. C. Bi, X. Xie, K. B. Yin, Y. L. Zhou, S. Wan, R. S. Ruof and L. T. Sun, J. Mater. Chem. A, 2014, 2, 1652 RSC.
-
(a) Q. Zhu and Q. M. Pan, ACS Nano, 2014, 8, 1402 CrossRef CAS PubMed;
(b) A. Bayat, S. F. Aghamiri, A. Moheb and G. R. Vakili-Nezhaad, Chem. Eng. Technol., 2005, 28, 1525 CrossRef CAS;
(c) T. R. Annunciado, T. H. D. Sydenstricker and S. C. Amico, Mar. Pollut. Bull., 2005, 50, 1340 CrossRef CAS PubMed;
(d) M. M. Radetic, D. M. Jocic, P. M. Jovanccic, Z. L. Petrovic and H. F. Thomas, Environ. Sci. Technol., 2003, 37, 1008 CrossRef CAS PubMed.
-
(a) X. C. Gui, J. Q. Wei, K. L. Wang, A. Y. Cao, H. W. Zhu, Y. Jia, Q. K. Shu and D. H. Wu, Adv. Mater., 2010, 22, 617 CrossRef CAS PubMed;
(b) H. C. Bi, X. Xie, K. B. Yin, Y. L. Zhou, S. Wan, L. B. He, F. Xu, F. Banhart, L. T. Sun and R. S. Ruoff, Adv. Funct. Mater., 2012, 22, 4421 CrossRef CAS;
(c) S. J. Choi, T. H. Kwon, H. Im, D. IlMoon, D. J. Baek, M.-L. Seol, J. P. Duarte and Y. K. Choi, ACS Appl. Mater. Interfaces, 2011, 3, 4552 CrossRef CAS PubMed;
(d) N. T. Cervin, C. Aulin, P. T. Larsson and L. Wagberg, Cellulose, 2012, 19, 401 CrossRef CAS;
(e) K. H. Kim, Y. G. Oh and M. F. Islam, Nat. Nanotechnol., 2012, 7, 562 CrossRef CAS PubMed;
(f) H. Hu, Z. B. Zhao, W. B. Wan, Y. Gogotsi and J. H. Qiu, Adv. Mater., 2013, 25, 2219 CrossRef CAS PubMed;
(g) X. Xie, Y. L. Zhou, H. C. Bi, K. B. Yin, S. Wan and L. T. Sun, Sci. Rep., 2013, 3, 2117 Search PubMed.
- L. Qiu, J. Z. Liu, S. L. Y. Chang, Y. Z. Wu and D. Li, Nat. Commun., 2012, 3, 1241 CrossRef PubMed.
-
(a) H. Y. Sun, Z. Xu and C. Gao, Adv. Mater., 2013, 25, 2554 CrossRef CAS PubMed;
(b) Z. Xu, H. Y. Sun and C. Gao, APL Mater., 2013, 1, 030901 CrossRef.
- S. Kabiri, D. N. H. Tran, T. Altalhi and D. Losic, Carbon, 2014, 80, 523 CrossRef CAS.
-
(a) Z. Xu, H. Y. Sun, X. L. Zhao and C. Gao, Adv. Mater., 2013, 25, 188 CrossRef CAS PubMed;
(b) L. Peng, Y. C. Zheng, J. C. Li, Y. Jin and C. Gao, ACS Catal., 2015, 5, 3387 CrossRef CAS.
- X. Li, P. X. Huang, Y. Zhou, H. Peng, W. Li, M. Z. Qu and Z. L. Yu, Mater. Lett., 2014, 133, 289 CrossRef CAS.
-
(a) D. N. H. Tran, S. Kabiri, T. R. Sim and D. Losic, Environ. Sci.: Water Res. Technol., 2015, 1, 298 RSC;
(b) M. Sun, Q. Tang, T. Zhang and G. Wang, RSC Adv., 2014, 4, 7774 RSC;
(c) C. Yang, J. Shen, C. Wang, H. Fei, H. Bao and G. Wang, J. Mater. Chem. A, 2014, 2, 1458 RSC.
- W. Chen and L. Yan, Nanoscale, 2011, 3, 3132 RSC.
- Z. Sui, Q. Meng, X. Zhang, R. Ma and B. Cao, J. Mater. Chem., 2012, 22(18), 8767 RSC.
-
(a) L. Qian and H. F. Zhang, J. Chem. Technol. Biotechnol., 2011, 86, 172 CrossRef CAS;
(b) H. C. Bi, K. B. Yin, X. Xie, Y. L. Zhou, N. Wan, F. Xu, F. Banhart, L. T. Sun and R. S. Ruoff, Adv. Mater., 2012, 24, 5124 CrossRef CAS PubMed.
- X. C. Dong, J. Chen, Y. W. Ma, J. Wang, M. B. Chan-Park, X. M. Liu, L. Wang, W. Huang and P. Chen, Chem. Commun., 2012, 48, 10660 RSC.
-
(a) B. Lee, S. Lee, M. Lee, D. H. Jeong, Y. B. Baek, J. Y. Yoon and Y. H. Kim, Nanoscale, 2015, 7, 6782 RSC;
(b) X. P. Zhang, D. Liu, L. Yang, L. M. Zhou and T. Y. You, J. Mater. Chem. A, 2015, 3, 10031 RSC.
Footnote |
† Electronic supplementary information (ESI) available: SEM images, TEM images and FT-IR spectra of the GCAs, as well as the schematic diagram of mechanical compression of the GCAs; movie of the mechanical strength test, movie of the absorption–combustion test, and movie of the adsorption–squeezing test. See DOI: 10.1039/c5en00125k |
|
This journal is © The Royal Society of Chemistry 2016 |