DOI:
10.1039/C6CC06475B
(Feature Article)
Chem. Commun., 2017,
53, 20-44
Beyond methylammonium lead iodide: prospects for the emergent field of ns2 containing solar absorbers
Received
5th August 2016
, Accepted 29th September 2016
First published on 29th September 2016
Abstract
The field of photovoltaics is undergoing a surge of interest following the recent discovery of the lead hybrid perovskites as a remarkably efficient class of solar absorber. Of these, methylammonium lead iodide (MAPI) has garnered significant attention due to its record breaking efficiencies, however, there are growing concerns surrounding its long-term stability. Many of the excellent properties seen in hybrid perovskites are thought to derive from the 6s2 electronic configuration of lead, a configuration seen in a range of post-transition metal compounds. In this review we look beyond MAPI to other ns2 solar absorbers, with the aim of identifying those materials likely to achieve high efficiencies. The ideal properties essential to produce highly efficient solar cells are discussed and used as a framework to assess the broad range of compounds this field encompasses. Bringing together the lessons learned from this wide-ranging collection of materials will be essential as attention turns toward producing the next generation of solar absorbers.
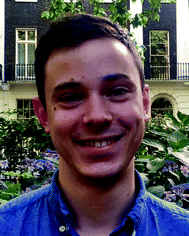
Alex M. Ganose
| Alex Ganose received his MSci (2014) in Natural Sciences and MRes (2015) in Molecular Modelling and Materials Science from University College London, UK. He is currently studying towards his EngD in Molecular Modelling and Materials Science under Dr David Scanlon at University College London. His research is focused on the computational design of earth-abundant and non-toxic solar absorbers. |
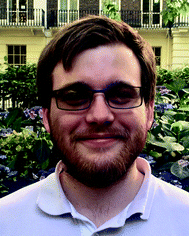
Christopher N. Savory
| Chris Savory received his MChem degree from the University of Oxford in 2014. He is now working towards his PhD in Inorganic Chemistry under the supervision of Dr David Scanlon at University College London. His research interests include the ab initio computational investigation of hybrid inorganic–organic, silver and bismuth materials for photovoltaic and other renewable energy applications. |
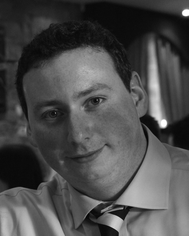
David O. Scanlon
| David O. Scanlon received his degree in Computational Chemistry (2006) and PhD in Chemistry (2011) from Trinity College Dublin, Ireland. After a Ramsay Fellowship in the Department of Chemistry at UCL, he was appointed to a Lectureship (2013) and then as a Reader (2016) in Computational, Inorganic and Materials Chemistry at UCL and at Diamond Light Source. He currently leads the Materials Theory Group, which focusses on the use of Computational Chemistry techniques to understand and predict the behaviours of solid state materials, primarily for renewable energy applications. The group is currently working on novel materials for photovoltaics and photocatalysis, Li-ion batteries, thermoelectrics, and optimising materials for thin film displays. |
1 Introduction
As modern society continues to consume natural resources at an ever-increasing rate, there is a growing demand for a clean energy source capable of providing indefinite and sustainable economic growth.1 Arguably the most abundant renewable energy resource is sunlight, with over 1500 exawatt-hours of energy falling incident on the earth in the form of solar radiation each year.2 The enormity of this resource is apparent when one considers that the total known reserves of oil, gas and coal amount to just 9 exawatt-hours. A year's worth of sunlight, therefore, provides almost two hundred times the energy of the world's entire known supply of fossil fuels.3 Of the technologies available to harness solar radiation, photovoltaic cells are perhaps the most promising due to their ability to convert light directly into electricity. Indeed, coverage of less than 0.3% of the earth's surface would be sufficient to meet the world's energy needs using the latest commercially available solar panels. However, in order for photovoltaic cells to compete with fossil fuels in utility-scale power generation, it is necessary to reduce the total cost of solar energy, either through increased efficiencies or lower cost per photovoltaic cell.4
The current photovoltaic market is dominated by crystalline silicon solar cells, which, having benefited from six decades of research, possess power conversion efficiencies (PCEs) around 21%.5 However, the performance of these cells is limited by the indirect band gap of silicon and the last five years have seen little improvement to their efficiencies. Currently, the highest performing single-junction solar cells are those containing GaAs. These devices have shown efficiencies approaching the Shockley–Queisser limit of 33%6 but due to the high raw materials cost of GaAs (∼7 times that of crystalline silicon),7 their viability is limited to extraterrestrial applications where efficiency presides over cost.8
Recently, hybrid halide perovskite solar cells have emerged as a serious contender to silicon-based devices thanks to an unprecedented rise in efficiency—reaching 22% in 2016, overtaking all other third-generation technologies.9,10 CH3NH3PbI3 (MAPI) is the champion hybrid halide perovskite absorber and has, as such, attracted an enormous volume of research interest. MAPI possesses many of the ideal properties for a solar absorber: a direct band gap of 1.55 eV,11 small exciton binding energies,12 high levels of defect self-regulation,13 remarkably long charge carrier diffusion lengths,14,15 and excellent charge carrier mobilities.16–18 It can also be solution-processed allowing for low-cost fabrication and has been shown to perform well with a wide range of hole and electron contact materials, allowing for widespread application.19–21 However, the intrinsic long-term stability of the MAPI structure is poor22,23 and, despite considerable effort, remains a significant challenge facing the hybrid perovskite community.24–26
Many studies have tried to bypass the instability of MAPI by exploring alternative compounds within the perovskite composition space.27–29 However, attempts to replace methylammonium with other organic and inorganic cations outside of those already known in the literature has proved exceedingly challenging.30,31 Whilst the tolerance factor metric has traditionally been applied as a predictor of perovskite stability, it performs poorly across the range of known iodide perovskites.32,33 Recently, a revised tolerance factor method has been developed that takes into account the greater covalency seen in some metal–halide bonds and is able to accurately predict the stability of the majority of halide perovskites.34 Strikingly, the report suggests that only a handful of halide perovskites remain to be discovered, with most likely to possess band gaps unsuitable for photovoltaic applications. It is therefore essential, now more than ever, that the search for the next generation of solar absorbers be extended beyond the cubic perovskite motif.
The meteoric rise in the efficiency of MAPI has fuelled intense interest among a broad community of physicists, chemists, and engineers and has brought together the lessons learned in over 20 years of development of related dye-sensitised and organic photovoltaic cells.35,36 Brandt et al. have recently proposed several key properties likely to give rise to highly efficient and defect-tolerant solar absorbers, including a large dielectric constant, small effective masses, a valence band maximum composed of antibonding states, and high levels of band dispersion.37 Materials containing post-transition metals with an ns2 electronic configuration (i.e. an N-2 oxidation state) possess many of these properties due to their soft polarisability—leading to high Born effective charges—and large spin–orbit effects, which act to increase the bandwidth of the conduction band.38,39 As such, a wide range of compounds comprising Pb2+, Sn2+, Ge2+, Sb3+, and Bi3+ cations are currently of interest for their solar absorber ability.
In this Review we focus on this emerging field of ns2 containing solar absorbers. The ideal properties needed to produce highly efficient solar cells are discussed and used as a framework to assess the broad range of compounds this field encompasses. Initially, group 14-based materials—those containing lead, tin and germanium—are examined, with both well established and novel absorbers considered. The second half of this Review concerns materials containing the group 15 post-transition metals antimony and bismuth. Throughout, particular attention is given to the relationship between structure and properties, specifically the effect of dimensionality on stability and carrier transport. Lastly, we look towards the future of next generation solar absorbers.
2 Desired solar absorber properties
The performance of novel solar absorbers is hard to predict in practice, due to the dependence on many external conditions such as the method of deposition, quality of precursor and device architecture. However, analysis of high performance solar materials reveals several key properties likely to beneficially affect device efficiencies.37 Crucially, many of these properties, whilst difficult to measure experimentally, can be obtained relatively cheaply from theoretical methods, thus highlighting the importance of a combined theoretical/experimental approach in screening new materials.
2.1 Magnitude and nature of the band gap
Arguably the most important property of a solar absorber is its band gap, as it determines the maximum theoretical PCE possible for the material. The best performing absorbers possess band gaps in the 1.10–1.55 eV range, as quantified by the well-known Shockley–Queisser limit,6 which takes into account the antagonistic dependence of short-circuit current (Jsc) and open-circuit voltage (Voc) on the band gap and solar spectrum (Fig. 1a). While this does not preclude materials with band gaps outside this range being examined, in order to maximise efficiency, a band gap close to 1.3 eV is highly advantageous.
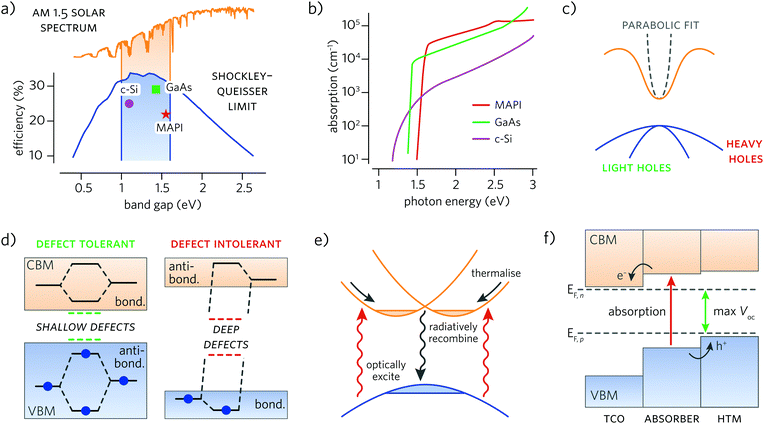 |
| Fig. 1 Desired solar absorber properties: (a) ideal range of solar absorber band gaps (shaded) projected onto the AM1.5 solar spectrum (yellow) and the Shockley–Queisser limit (blue); (b) importance of a direct band gap transition—crystalline silicon (c-Si, purple) has dramatically weaker absorption relative to GaAs (green) and MAPI (red); (c) schematic band structure indicating how greater band dispersion (curvature) gives rise to smaller hole and electron effective masses; (d) the impact of bonding structure on defect tolerance, with antibonding states at the top of the valence band maximum giving rise to shallower defects; (e) schematic of absorption and recombination processes in Rashba spin-split systems; (f) band alignment in a heterojunction solar cell. Efficient alignment between materials results in a larger maximum obtainable open circuit voltage (Voc). The Fermi level of the n-type transparent conducting oxide (TCO) and p-type hole transporting material (HTM) layers is denoted by EF,n and EF,p, respectively. In panels (c) and (e) conduction bands and valence bands are shown in orange and blue, respectively; (b), (d), and (e) adapted with permission from ref. 43–45. | |
2.2 Strength of optical absorption
Strong optical absorption is particularly crucial for solar absorbers: many compounds with ideal band gaps are poor absorbers. Indeed, low absorbance due to an indirect band gap is one of the primary reasons to look beyond crystalline silicon as an absorber. As recently stressed by Yu and Zunger, strong absorption requires a direct band gap transition; however, materials with indirect band gaps may still perform well if a direct transition of suitable energy is also available.40 Loss in absorption can further result if the fundamental band gap is dipole disallowed, widening the optical band gap relative to the fundamental gap, as often results in centrosymmetric materials.41,42 Ideally, strong absorption is characterised by a steep absorption edge in the absorption coefficient, α, just above the band gap, up to 104–105 cm−1 or higher (Fig. 1b).43
2.3 Charge carrier effective mass
High charge carrier mobilities can be particularly useful in photovoltaics for establishing electron–hole separation and improving device performance. Mobility, μ, is dependent on the dispersion of the band edges in a material—theoretically quantified by the effective mass of a carrier—with greater dispersion giving rise to smaller effective masses and in turn, enhanced carrier mobilities (Fig. 1c). The primary limiter of carrier mobility is through scattering by defects, phonons and other charge carriers. We note that mobility is not the only important transport property: minority-carrier lifetimes, τ, have recently been proposed as an essential metric for screening novel PV materials, due to their role in Shockley–Read–Hall recombination (trap-assisted non-radiative recombination).37,46,47 Indeed, MAPI's excellent performance is dependent on both its high mobilities and very long carrier lifetimes and diffusion lengths.17,48
2.4 Defect tolerance
Defect tolerance is the ability for semiconductors to retain strong optoelectronic properties, particularly power conversion efficiency, regardless of the presence of defects, including point defects and grain boundaries.49 One of the proposed mechanisms by which defect tolerance may occur is the presence of antibonding interactions at the valence band maximum (Fig. 1d)—as a result, defects are confined to shallow states at the band edges, rather than deep gap states that may act as traps and recombination centres.44 As Brandt et al. recently highlighted, ions such as Sn2+ and Bi3+ are highly likely to have this bonding composition due to the active ns2 lone pair, and so present excellent candidates for defect tolerant compounds.37
2.5 Dielectric constant and ferroelectric behaviour
Electric response can also be vital to a photovoltaic absorber. A large static dielectric constant has perhaps the most obvious benefit, particularly with regard to some of the aforementioned properties—it confers a high degree of charge screening, resulting in smaller defect charge-capture cross-sections, and inhibits radiative electron–hole recombination. Furthermore, for ‘hydrogenic’ defects, a large dielectric constant enables smaller defect binding energies promoting shallower defect states.50 As with defect tolerance, large highly polarizable cations like Pb2+ are likely to lead to high dielectric properties. Ferroelectric behaviour has also been of considerable interest with regards to MAPI's hysteresis;38,51,52 while current evidence suggests it may not be the primary cause of hysteresis,53 the prospect of useable photoferroic devices, and high photovoltages from the anomalous photovoltaic effect, remains enticing.16,54
2.6 Rashba splitting
‘Spintronics’ is an emergent field in condensed matter physics, of which MAPI has seen its share of interest. Recently, Rashba splitting in the MAPI electronic band structure has been implicated in strongly reducing radiative recombination and is considered a possible cause of its high carrier lifetimes.55,56 Indeed, the spin-split indirect gap seen in MAPI is thought to reduce the recombination rate by a factor of more than 350% compared to direct band gap behaviour (Fig. 1e).45 Non-centrosymmetric structures with heavy elements such as bismuth and lead could easily demonstrate similar effects due to due their strong spin orbit coupling. Likewise, multivalley band structures are also thought to increase charge carrier mobilities through separation of charge carriers, in addition to ensuring a high density of states at the band edges, leading to higher absorption coefficients.40,57
2.7 Alignment with commonly used contact materials
During heterojunction cell construction, care must be taken to ensure close band alignment of the absorber with its neighbouring materials, such as buffer layers and contacts materials. Efficient band alignment prevents loss of Voc and enables facile carrier transport throughout the cell (Fig. 1f). While layers can be tuned to ensure this, an absorber with typical band positions that align well with ubiquitous components like F-doped SnO2 would be highly advantageous to reducing the cost and difficulty of manufacture and distribution.
3 Lead absorbers
3.1 Perovskite structured
As previously mentioned, stability is a major concern limiting the use of hybrid halide perovskites in commercial photovoltaic devices, primarily as longevity is crucial to reach energy payback times.58 Many studies have focused on moisture degradation of MAPI cells,59,60 highlighting the facile hydrolysation of the absorbing layer upon contact with air.61–63 Additionally, MAPI possesses poor thermal stability and is known to rapidly decompose at temperatures above 85 °C,64,65 with research indicating that the MAPI structure is intrinsically unstable with respect to phase separation into CH3NH3PbI3 and PbI2.25,66 This instability has recently been attributed, with the aid of computational studies, to the low formation energy of MAPI.67,68 As such, modifications of the MAPI formula that are able to increase stability have become highly desirable.69
One method of tuning MAPI's electronic properties is through changing the organic cation (Fig. 2a). So far, only methylammonium (MA) and formamidinium (FA) have been successfully incorporated into the perovskite structure, with larger cations resulting in lower dimensionality structures due to disruption of the three-dimensional (3D) Pb–I cage.31,70,71 Replacing MA with FA to yield CH(NH2)2PbI3 (FAPI), results in films with a slightly smaller band gap of 1.48 eV, long photoluminescence (PL) lifetimes, lower rates of recombination, high PCEs, and enhanced thermal stability.72,73 Unfortunately, the synthesis of FAPI is complicated by the formation of a thermally accessible hexagonal δ-phase, whose large band gap adversely affects device performance.74,75 FAPI films incorporating up to 20% MA show considerable stabilisation of the black α-phase during synthesis and possess long exciton lifetimes and high efficiencies,76,77 however, the long term stability of these films has not yet been addressed. Alternatively, replacing the organic component of MAPI with an inorganic cation to produce an all-inorganic perovskite is considered a possible route to enhanced stabilities.78,79 Eperon et al. have recently demonstrated a working CsPbI3 based device by preventing the formation of the weakly absorbing yellow non-perovskite phase.80,81 Their cells showed remarkable thermal stability up to 300 °C but possessed poor efficiencies of only 2.9% and extreme sensitivity to ambient conditions.
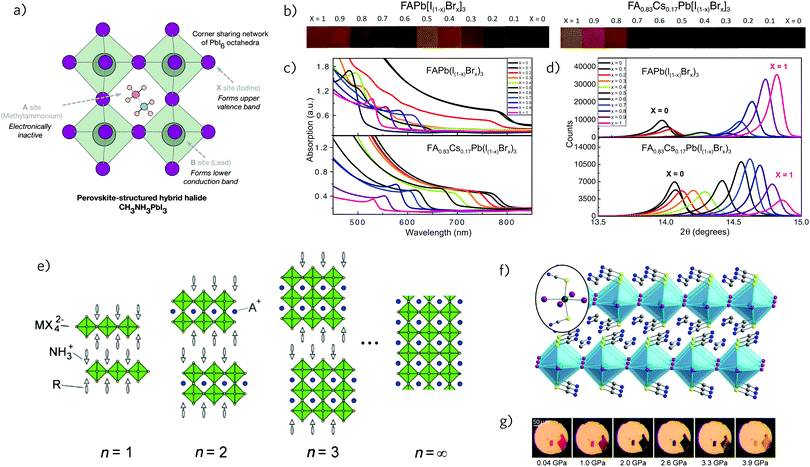 |
| Fig. 2 (a) Schematic of perovskite structure indicating the A, B, and X lattice sites. Reprinted with permission from ref. 90. Copyright 2015, American Chemical Society. (b–d) Photographs (b), ultraviolet-visible absorbance spectra (c) and X-ray diffraction patterns (d) of FAPb[I(1−x)Brx]3 and FA0.83Cs0.17Pb[I(1−x)Brx]3 perovskite films, with Br composition increasing from x = 0 to 1. From ref. 89. Reprinted with permission from AAAS. (e) Layered 〈100〉-oriented perovskite structures, where n = ∞ denotes the cubic 3D perovskite, n = 1 corresponds to the 2D layered structure, and n > 1 describes “quasi-2D” structures. Reprinted with permission from ref. 70. (f) Crystal structure and (g) piezochromic response of (CH3NH3)2Pb(SCN)2I2 (MAPSI). Reprinted with permission from ref. 91. Copyright 2016, American Chemical Society. | |
MAPI can also be tuned through the replacement of iodide with other halides (Fig. 2a).82 In many early cells, using a PbCl2 precursor incorporated a very small proportion of the chlorine, leading to improved film morphologies due to better distributed heterogeneous nucleation.48,83,84 On the other hand, inclusion of bromide in a solid solution, allows for a tunable band gap85 and lower levels of hysteresis.86 Based on this Rehman et al. evaluated the mixed Br/I system, FAPb(BrxI1−x)3, for use in tandem solar cells.87 It was noted that the composition needed to form an ideal top-cell band gap of ∼1.7–1.8 eV (namely x = 0.3–0.5), resulted in apparently “amorphous” phases with reduced charge-carrier diffusion lengths, high levels of energetic disorder, and poor optical absorption. This is also been observed in theoretical calculations of the MAPb(BrxI1−x)3 system, in which the region between 0.3 < x < 0.6 is unstable with respect to spinodal decomposition at 300 K.88 Recently, McMeekin et al. have demonstrated a mixed-cation mixed-halide perovskite system, in which the phase instability region is subjugated through partial substitution of FA with Cs (Fig. 2b–d).89 The resulting thin films, with the composition FA0.83Cs0.17Pb(I0.6Br0.4)3, possessed excellent crystallinity, an optical band gap of 1.74 eV, a high open circuit voltage (VOC) of 1.2 eV and efficiencies competitive with those seen in MAPI based solar cells (up to 17.9%). The same composition was employed as a semitransparent cell along with a crystalline silicon module, in order to assess the potential of these films for tandem architectures, with an efficiency of 19.8% reported.
3.2 Reduced dimensionality perovskites
Layered perovskites have recently become of interest as a route to increased stabilities.92 As previously discussed, incorporating large organic cations in the synthesis of MAPI results in lower dimensionality structures, where the cations cannot fit within the perovskite cage.32,33 In these structures, the large cations are sandwiched between slabs of PbI6 octahedra, effectively “capping” the perovskite layers.93 The resulting two-dimensional (2D) structures, or Ruddlesden–Popper like phases, appear to be stabilised by van der Waals interactions between the capping molecules.94–97 Similar to MAPI, the physical and structural properties of these compounds can be fine tuned through tailoring of the organic cation, halide and metal components.31,70 By adjusting the stoichiometric quantities of the bulky organic cation versus lead iodide (PbI2) and methylammonium iodide (CH3NH3I, MAI), the number of layers of perovskite octahedra in each slab (n) for the series PEA2(CH3NH3)n−1PbnI3n+1, can be controlled.98 In this way, the limit n = ∞ represents a cubic 3D perovskite, n = 1 corresponds to a 2D layered structure and n > 1 describes “quasi-2D” perovskite structures (Fig. 2e).
A range of intercalated organic–inorganic lead, tin, and germanium perovskites were first structurally characterised in the 1990's by Mitzi et al., who noted the role of the large cations in aiding thermal and chemical stability, as well as influencing optical and electronic properties.93–95,98–104 Generally, upon moving from the 3D perovskite structure to the 2D n = 1 phase, the optical band gap widens considerably and n-type conductivity decreases. This is partially a result of the high exciton binding energies (often >300 eV)101,105 seen in these structures, which arise due to spatial restrictions and dielectric mismatch between the organic and inorganic layers.106,107 In the context of solar cells, this combination of properties is undesirable as it limits Voc and is likely to result in impaired performance. Stability, on the other hand, is found to be highest for the 2D structures and decrease with increasing values of n. As such, the primary challenge presented by reduced dimensionality perovskites is to optimise stoichiometry, so as to balance stability against electronic and optical performance.
One route to producing suitable 2D perovskites is through introducing enhanced halogen or hydrogen bonding at the organic–inorganic interface, which results in a red shifting of the band gap due to a more disperse valence band maximum.108,109 The first layered perovskites put to use in solar cell applications adopted a different approach, instead tuning the band gap through judicious choice of organic cation along with careful stoichiometric control. Smith et al. employed bulky C6H5(CH2)2NH3+ (PEA) as the organic cation, producing films with 3 perovskite layers (n = 3), an optical band gap of 2.1 eV and reasonably small exciton binding energies (40 meV).110 Their cells displayed efficiencies up to 4.73% and were stable after 40 days in air with 52% humidity. Similarly, Cao et al. produced n = 3 devices containing CH3(CH2)3NH3+via a simple one-step spin-coating process, that exhibited strong light absorption, smooth film surfaces and efficiencies of 4.02%.111
The initial attempts at producing 2D perovskite devices suffered from poor charge carrier extraction that limited their efficiencies.110,111 Recently, Quan et al. have demonstrated the first certified hysteresis-free planar perovskite solar cell, containing a 2D absorbing layer.96 Using a combination of density functional theory (DFT) calculations and complementary studies on physical and optoelectronic properties, the number of perovskite layers was optimised to 40 < n < 60. As expected, van der Waals interactions between the PEA molecules were found to drive increased stability, with the forces acting to reduce the desorption rate of MAI (a key decomposition pathway in MAPI)112–114 by 6 orders of magnitude. Interestingly, the trend in optical band gap (decreasing with increasing n)93 was found to result from a gradual shift in the conduction band minimum, with the valence band maximum effectively fixed in energy. The resulting quasi-2D devices combined the stability of 2D perovskites with the excellent charge-carrier transport and optical properties of MAPI, resulting in a PCE of 15.3% for the composition where n = 60.96 Another recent paper has succeeded in producing layered perovskite films orientated such that the sheets form perpendicular with respect to the substrate.115 This further motivates the development of layered perovskite as it enables clear carrier extraction pathways from the absorber layer to the device contacts.
The last year has seen the substitution of iodine for thiocyanate (SCN−) emerge as an avenue to improving the stability of MAPI whilst retaining high efficiencies.71,116,117 Inclusion of small amounts of SCN−, a pseudo-halide with an ionic radius similar to that of I− (217 pm vs. 220 pm),118 has been shown to produce cells with larger crystal sizes and fewer trap states.119 Furthermore, the reaction of MAI and Pb(SCN)2 produces the compound (CH3NH3)2Pb(SCN)2I2 (MAPSI),120 which is similar in structure to the n = 1 layered perovskites but with Pb2+ octahedrally coordinated to four equatorial I− and two axial SCN− ions (Fig. 2e).121 A debate exists as to the size of MAPSI's optical band gap: several studies have reported a direct optical gap of 1.57 eV,119,121 however a recent paper by Xiao et al. demonstrated a thin film possessing direct and indirect optical band gaps of 2.04 eV and 2.11 eV, respectively.122 Similarly, Umeyama et al. also report a larger band gap for MAPSI, noting a red-to-black piezochromic response upon compression (Fig. 2g).91 The exact cause of this discrepancy has not yet been elucidated, however it has been suggested that contamination of MAPSI with MAPI may play a role. Similar to other 2D perovskites, the layered structure results in an increased enthalpy of formation, bestowing MAPSI with increased chemical stability.68 Devices containing MAPSI have recorded efficiencies up to 8.3%, with a larger Voc and enhanced stability in air with 95% humidity than comparable MAPI films.120 Additionally, it should be possible to tune MAPSI through incorporating other pseudo-halides (such as OCN and SeCN), halides (Cl, Br, I), and metals (Sn, Ge), opening up the possibility for a range of MAPSI structured analogues.68
3.3 Photoactive organic cations
When the size of the organic cation is increased further still, interconnectivity of the lead iodide octahedral becomes difficult due to large steric effects, and zero-, one-, or two-dimensional (0D, 1D, or 2D) structures result.104,123 These topologies, characterised by clusters (0D), nanowires (1D), and corrugated sheets (2D) often possess excellent stability but suffer from wide band gaps and poor charge carrier mobilities, due to the lack of clear conduction pathways within the crystal.124–127 An emerging route to overcoming such properties is the use of photoactive organic cations, primarily those containing aromatic moieties, which can contribute to the optical response of the material.128,129 Here, shrewd choice of cation is essential to ensure a significant interaction between the organic and inorganic components, and result in broad band spectral absorption.130,131
Maughan et al. have recently employed the aromatic cycloheptatrienyl ([C7H7]+, tropylium) ion128 due to its strong absorption, electronic sensitivity (allowing for optical fine-tuning) and chemical stability, which make it likely to interact favourably with an inorganic system.132,133 The resulting compound, C7H7PbI3, forms a nanowire-type structure composed of edge-sharing PbI6 octahedra, with the tropylium ions distributed between the wires (Fig. 3a). DFT calculations show that the band gap results from charge transfer between the inorganic and organic components, however, its band gap of 2.15 eV is too large for a high efficiency solar absorber. A similar system has been demonstrated using a significantly bulkier organic ligand, N,N′-di(4-pyridyl)-1,4,5,8-napthalene diimide (DPNDI), in which nanowires of face-sharing lead iodide octahedra are encased by an interwoven network of protonated DPNDI molecules (Fig. 3b).134 Beneficial hydrogen bonding and anion–π interactions act to reduce the distance between the ligand and nanowire, enabling a material with an ideal band gap of 1.27 eV and unusually long-lived charge-separated states. The efficient charge-carrier separation is expected to lead to reduced recombination losses, however, the loss in dimensionality results in high effective masses and therefore is likely to adversely affect electron mobilities.135
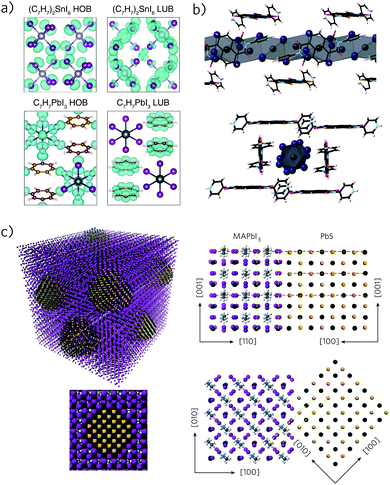 |
| Fig. 3 (a) Band decomposed charge densities of tropylium tin iodide and tropylium lead iodide, indicating the highest occupied band (HOB) and lowest unoccupied band (LUB) for each material. Reprinted with permission from ref. 128. Copyright 2015, American Chemical Society. (b) (Pb2I6)·(H2DPNDI)·(H2O)·(NMP) viewed along the a and b axes, illustrating the 1D lead iodide nanowires and DPNDI network. Reprinted with permission from ref. 135. (c) Quantum-dot-in-perovskite model. Favourable lattice matching between the PbS quantum dot and lead iodide perovskite allows for efficient heteroepitaxial growth in both the X–Y and X–Z planes. Reprinted with permission from ref. 138. Copyright 2015, Nature Publishing Group. | |
Materials comprising mixed inorganic and organic connectivity have been suggested as one method of countering poor mobilities. By designing systems in which charge carriers are mobile in both the inorganic network along with an overlapping network of π-stacked photoactive organic ligands, multiple pathways for carrier extraction can be developed.136 This has recently been realised in systems containing 1D lead iodide chains coordinated to large aromatic dipyrido- and benzodipyrido-phenazine compounds, which form eclipsed channels connecting the inorganic chains.137 It was found that photoexcitation effects charge transfer from the lead iodide component to the organic moiety, however the large band gaps of the materials (2.13 eV and 2.52 eV) prevents their use in photovoltaic applications. While it is clear that this system highlights the viability of the technique, further optimisation of the organic component to produce the desired optical response and structure directing effects is essential.
3.4 Lead(II) chalcogenides
PbS is a quintessential lone pair absorbing material, having been studied for over a decade for use in quantum dot (QD) sensitized solar cells due to its high absorption coefficient, stability in air and ability to generate and split multiple excitons.139–143 Furthermore, PbS possesses a large Bohr exciton radius of 18 nm, enabling QDs with excellent charge carrier mobilities and a highly tunable band gap.144,145 The band alignment of PbS QDs can be engineered through the use of different ligand treatments146,147 and has enabled rapid advancement in PbS solar cells, with a maximum certified efficiency of 9.2%.148 PbS has also been employed in a core/shell configuration with CdS, which aids surface passivation and has enabled efficiencies up to 5.6% with an enhanced open circuit voltage of 0.66 V.149
The highly tunable band gap of PbS (from 0.7 eV to 1.5 eV) means it is possible to produce QDs that absorb in the near- or mid-infrared portion of the solar spectrum, which, when combined with hole conducting polymers that absorb in the UV-visible range, allows for broad spectral coverage.150 Lu et al. produced PbS polymer:nanocrystal composites, based on a donor–acceptor polymer, leading to efficiencies of 4.8% with efficient charge separation from PbS to the polymer and enhanced colloidal stability.151 PbS has similarly been employed in perovskite solar cells, functioning simultaneously as a co-sensitiser and hole transporting material, enabling cells with a panchromatic response, high photocurrent densities up to 24 mA cm−2 and efficiencies of 3.6%, despite low open circuit voltages of 0.34 V.152 By tuning the band alignments of the PbS QDs, a dramatically increased VOC of 0.86 V was achieved, resulting in cells with a PCE of 7.5%.153 Remarkably, due to favourable lattice matching, preformed PbS quantum dots have been incorporated whole into the MAPI structure, producing epitaxially aligned “dots-in-a-matrix” crystals (Fig. 3c).138 The resulting heterocrystals possessed a modulated optical response, which, when decomposed, indicated that the properties of the QDs and perovskite matrix remained otherwise unaltered. However, the small band gap of the QDs employed (1 eV) and the highly efficient transfer of photo-excited charge carriers from the perovskite to the PbS nanocrystals, while useful for infra-red applications, limits the applicability of the system with regard to solar power generation.
The closely related compounds, PbSe and PbTe, are also of intense interest as a quantum dot solar absorbers, partly due to their even larger Bohr radii of 46 nm154 and 152 nm,155 which when coupled with well established and finely size-tunable synthesis methods, enables precise control over the band gap from 0.4–1.4 eV.156 Pb-based QD solar cells are additionally attractive due to their enhanced stability in air when compared to other solar absorbers. Indeed, the maximum recorded efficiency for a PbSe-based QD solar cell of 6.2% was obtained in devices fabricated in an ambient atmosphere, demonstrating the stability of these devices.157 Furthermore, previous studies on PbS have demonstrated its stability in air over 1000 hours of light illumination without device encapsulation.158 As such, it is clear that lead salts such as PbS and PbSe have an important role to play in the future of colloidal quantum dot photovoltaics. The low cost of raw materials7 combined with their significant versatility guarantees that Pb-based quantum dot cells will remain a highly studied class of materials.
4 Tin absorbers
Lead-based photovoltaics, while generally the highest performing ns2 solar absorbers, have come under significant scrutiny due to concerns over the toxicity of lead.159 It is important to note that within the EU, commercial solar cells are exempt from the Restriction of Hazardous Substances Directive and, as such, are not subject to regulation over their lead content.160 Regardless, the intrinsic instability of MAPI64 presents the risk that toxic material may be released into the environment unless devices are sufficiently encapsulated, necessitating an increase in the cost of device fabrication.59,161,162 The last few years have therefore seen a concerted effort to eliminate the use of lead in photovoltaic devices. In this regard, tin is of considerable interest as it is both cheap and non-toxic,163 and, being a group 14 metal, is expected to show much of the same coordination chemistry and electronic properties seen in the lead analogues. Consequently, materials containing tin have long been sought after as a route to an earth-abundant and non-toxic solar absorber.
4.1 Perovskite structured
Due to the successes of the lead hybrid perovskites there has been a significant research effort towards synthesising and evaluating the tin analogues. Unfortunately, the propensity for Sn2+ to undergo oxidation to Sn4+ has proved challenging to overcome and still represents a major decomposition mechanism in the tin hybrid perovskites.39,167 Initially, partial substitution of Pb with Sn to form CH3NH3Sn0.25Pb0.75 enabled a reduction in band gap relative to MAPI, with devices showing efficiencies up to 7.37%.168,169 In an attempt to counteract the low photocurrent densities resulting from poor film coverage, PbCl2 was added to produce planar CH3NH3SnnPb1−nI3−xClx based cells with 10.1% efficiency.170 In 2016, mixed Pb–Sn perovskite films with the composition CH3NH3Sn0.5Pb0.5I3 achieved efficiencies of 13.6%, demonstrating the potential of devices with reduced lead content to achieve high performance.171
It was originally thought that the presence of Pb was essential to prevent undesirable oxidation of Sn, however, in 2014, two groups were successful in yielding solar cells based on the completely lead-free CH3NH3SnI3.172,173 These devices, with efficiencies of 5.23%172 and 6.4%,173 were made possible through rigorous glovebox protocols and ultra-high purity starting materials. CH3NH3SnI3 possesses an optical band gap of 1.30 eV39,74 and, similarly to MAPI, is intrinsically p-type, with relatively high carrier densities (1019 cm−3).174 Devices based on a CH3NH3SnI3/SiO2 nanocomposite architecture have shown impressive open circuit voltages up to 0.88 V, only 350 mV less than the thermodynamic limit.173 However, small carrier diffusion lengths (30 nm) along with poor long-term stability present a significant obstacle to overcome before these devices can find practical applications.90
In 2015, Koh et al. revealed that inclusion of SnF2 in the synthesis of formamidinium tin iodide (HC(NH2)2SnI3, FASI) counteracts the facile oxidation of tin by promoting the reduction of Sn4+ to Sn2+, however, this increased resilience to oxidation occurs at the cost of decreased conductivity.175 This technique enabled, for the first time, the production of FASI-based solar cells with power conversion efficiencies up to 2.1%. FASI has an ideal band gap of 1.4 eV and, unlike the analogous lead compound, FAPI, does not possess any other thermally accessible phases that can cannibalise device performance.74,77,176 Indeed, the stability of FASI has been demonstrated up to 200 °C, well above maximum device operating temperatures.175 Recently, this technique has been extended to prevent phase segregation on the surface of the films through the formation of a SnF2–pyrazine complex, yielding devices with power conversion efficiencies of 4.8% (Fig. 4a).164 These films displayed smoother surface morphologies and exhibited longer recombination lifetimes than reference cells prepared in the absence of pyrazine. Impressively, encapsulated devices stored in ambient conditions showed stable performance for over 100 days, with a loss in efficiency of only 2% over this period. Currently, however, a combination of low open circuit voltage (0.32 V) and fill factor (63%) prevent these devices from achieving performance comparable with other perovskite technologies.164
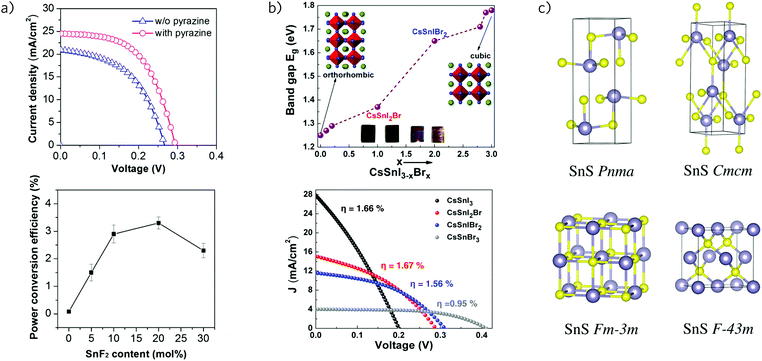 |
| Fig. 4 (a) J–V curves and trend in band gap demonstrating the effect of pyrazine and concentration of SnF2 of FASnI3 perovskite solar cells. Adapted with permission from ref. 164. Copyright 2016, American Chemical Society. (b) Trend in band gap and J–V curves for CsSnI3−xBrx perovskite solar cells, where x = 0 to 3. Adapted with permission from ref. 165. Copyright 2015, American Chemical Society. (c) Crystal structures of tin monosulfide. The lowest energy structure is herzenbergite (Pnma), a distorted rocksalt structure (Fm m). Several reports have identified tin monosulfide in the zincblende crystal structure (F 3m), however, DFT calculations reveal this phase to be high in energy. Adapted with permission from ref. 166. Copyright 2012, American Chemical Society. | |
The issue of unwanted oxidation has also plagued the all-inorganic perovskite, CsSnI3. First used as a solid-state hole transporting material in dye-sensitised solar cells,177 CsSnI3 is a p-type semiconductor with a band gap of 1.30 eV.178,179 The first planar heterojunction devices employing CsSnI3 as the absorber material demonstrated power conversion efficiencies up to 0.9%, but were inhibited by poor stability and the polycrystalline nature of the films.180 Subsequent studies have, similarly to the other tin perovskites, employed SnF2 as an additive to control the oxidation state of tin, enabling efficiencies of 2.0% and high photocurrents of 22.7 mA cm−2.165,181 In these cells, performance was severely restricted by low open circuit voltages of 0.24 V, about 4 times less than those seen in typical MAPI devices.182,183 This has been partially mediated through the substitution of iodine with bromine to form CsSnI3−xBrx, enabling higher open circuit voltages due to blue shifting of the band gap (Fig. 4b).165 Unfortunately, unlike in the lead hybrid perovskites where inclusion of bromine has been shown to increase stability in humid conditions,85,184 no such resistance is conferred in the case of CsSnI3, with the oxidation of Sn2+ to Sn4+ remaining the primary chemical instability. Additionally, alongside problems relating to unwanted oxidation, CsSnI3 also possesses an alternative 1D yellow phase, with a band gap of 2.6 eV, formed under exposure to air or organic solvents.178,185,186 As such, it is clear that significant work is needed to improve the stability of CsSnI3 based devices if they are to find practical applications.
4.2 Tin(II) chalcogenides
Tin(II) monosulfide (SnS) possesses many of the ideal properties for a highly efficient solar absorber, such as a high absorption coefficient greater than 104 cm−1 and carrier concentrations around 1017–1018 cm−3.187–191 However, despite these properties and over 20 years of active research,192 the efficiency of SnS-based devices has yet to reach 5%.193,194 Nonetheless, the abundance of tin and sulphur, combined with the ease of materials processing and a maximum predicted efficiency of 32% (as per the Shockley–Queisser limit),195 make SnS attractive as a candidate photovoltaic absorber. SnS possesses an indirect fundamental band gap of 1.1 eV,196–198 however, its optical absorption (onset at ∼1.3 eV) remains high due to its slightly larger direct band gap of 1.2–1.5 eV, suggesting that the indirect gap is not detrimental to performance.192,199–201 SnS is intrinsically p-type, with low hole effective masses (mh* = 0.2 m0 in the a and b directions; mh* = m0 along c) and is generally employed in a p–n junction architecture using ZnO or CdO as the n-type layer.190 Unlike PbS, the small Bohr radius of SnS (7 nm) precludes its use in quantum dot solar cells.202
Several distinct phases of tin(II) monosulfide are known,188,203–205 with the most stable room temperature structure being herzenbergite, a distorted rock salt structure (α-SnS, Pnma).166 The distortion of the lattice from a cubic to orthorhombic structure is due to the strong Sn 5s2 lone pair (following the revised lone pair model)206,207 which repel and result in the formation of a layered system held together by weak covalent interactions (Fig. 4c). This 2D structure is the source of the anisotropy seen in the hole effective masses.190 Thin films of SnS have been produced by a wide range of deposition techniques, including chemical bath deposition (CBD),208–210 atomic layer deposition (ALD),211,212 spray pyrolysis,213–215 and thermal evaporation,216,217 of which the latter two techniques are most common. Choice of deposition method can have a profound impact on the optoelectronic properties of the films, with CBD, ALD and vapor transport deposition generally producing films with the largest carrier concentrations and highly mobile holes.188,218 It should be noted that the variation in microstructure is large, even within the same deposition method, and is expected to be a primary factor in determining the efficiency of the films, due to the predominant formation of small structures with large grain boundaries between crystallites.219–222
Sinsermsuksakul et al. have reported the most efficient SnS-based solar cell to date, producing a cell with a PCE of 4.4%.193 Their device, along with the other highest performing SnS solar cells, employed Mo as the metallic contact and ZnO as the n-type transparent electrode, with the addition of an optimised Zn(O,S) buffer layer necessary to achieve high performance.223,224 Interestingly, Burton et al. have shown Mo to be unsuited as a back contact material due to the small ionisation potential of SnS (4.7 eV), instead suggesting metals with lower workfunctions, such as Ti, W, or Sn, as optimal contact materials.225 Other reports, based on impedance spectroscopy measurements, indicate Mo performs poorly due to increased series resistance and high rates of tunnelling assisted recombination and have recommended Cu as a viable alternative.226
There has been some controversy surrounding the reports of zincblende structured SnS (F
3mm) deposited as microparticles and thin-films (Fig. 4c).188,194,203,227 Such a material would allow for extended compatibility with existing II–VI and III–V tetrahedral semiconductor technologies due to favourable lattice matching at the interface between the absorber material and window layers. However, there have been few subsequent reports of SnS adopting the zincblende structure and theoretical calculations reveal the phase to be unusually high in energy, with Burton et al. proposing that such structures have been mis-assigned zincblende instead of distorted-rocksalt.166 Recently, Nair et al. have provided evidence for a cubic SnS structure which they suggest helps to characterise those structures previously assigned as zincblende.228 Based on a previous single-crystal electron diffraction report that demonstrated cubic SnS (P213),229 the authors have produced SnS thin films with a direct band gap of 1.66–1.72 eV that can be formed via a number of synthetic routes. Nair et al. have also reported an analogous cubic SnSe compound, with a direct optical band gap of 1.4 eV, considerably larger than the 0.95–1.11 eV indirect gap of the rocksalt SnSe structure, and in the ideal range for a highly efficient solar cell.230 As of yet these cubic phases have yet to be incorporated into functioning photovoltaic devices, however, development of these materials is of obvious interest to the field.
5 Germanium absorbers
5.1 Perovskite structured
Germanium, another group 14 metal, has received relatively little attention as a replacement to lead, in part due to its strong lone pair effect, which acts to produce lower dimensionality structures with poor conductivities. Regardless, germanium is non-toxic and relatively earth-abundant and is therefore an ideal candidate for novel solar absorbing materials.231 The series of perovskite-structured CsGeX3 compounds, where X is Cl, Br, and I, have been known for some time due to their nonlinear optical properties.232–235 They crystallise in a rhombohedral structure (space group R3m) with band gaps of 3.1 eV, 2.3 eV, and 1.6 eV for X = Cl, Br, and I, respectively.236,237 Interestingly, CsGeI3 does not possess any other thermally accessible phases and is stable up to 350 °C,238 with devices recording efficiencies up to 3.2%.239 However, the stability of Ge2+ is significantly reduced due the low binding energy of its 4s2 electrons, which increase its sensitivity to oxidation.90 As such, rigorous glovebox protocols and suitable device encapsulation are essential for efficient device performance.240
Recently, two groups have reported a range of germanium-based hybrid perovskite materials with properties suitable for solar cell applications (Fig. 5a).238,240 It was found that replacement of the alkali metal acts to increase the band gap in line with the size of the cation, from ∼1.9 eV and ∼2.2 eV in the case of CH3NH3GeI3 (MAGI) and HC(NH2)2GeI3 (FAGI), up to 2.8 eV when trimethylammonium was employed ((CH3)3NHGeI3).240,242 Incorporation of cations larger than formamidinium results in highly distorted structures due to the stereochemical activation of the 4s2 lone pair.240 This reduces the bandwidth of the frontier bands and leads to a significant widening of the band gap, resulting in semi-transparent materials with extremely poor absorption. The large band gaps seen in these materials are not expected to produce efficient standalone solar cells and are instead more relevant as top layer absorbers in tandem devices. Despite this, MAGI has been incorporated into a functioning photovoltaic device but showed poor performance due to low open circuit voltages (0.15 V) thought to result from formation of Ge4+, with a maximum efficiency of 0.2% obtained.238 Overall, if germanium perovskite solar absorbers are to succeed, significant development of chemical methods to prevent unwanted oxidation is essential. One possibility is through layered perovskite structures, which, analogously to the lead equivalents, may gain some resistance to oxidation through van der Waals interactions acting on the surface of the GeI6 octahedra.101
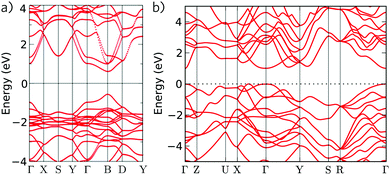 |
| Fig. 5 Band structures of germanium-based solar absorbers: (a) CH3NH3GeI3—adapted with permission from ref. 240. Copyright 2015, American Chemical Society—and (b) GeS. Adapted with permission from ref. 241, Copyright 2013, American Physical Society. | |
5.2 Germanium(II) chalcogenides
The germanium chalcogenides, GeS and GeSe, are also of interest as ns2 solar absorbers due to their ideal band gaps of 1.6–1.7 eV and 1.1–1.2 eV, respectively.241,244–246 Whilst both compounds, similarly to their tin analogues, possess indirect fundamental band gaps, their direct gaps are only slightly larger (∼0.08 eV) due band structures which contain multiple band extrema close in energy (Fig. 5b).247,248 Both are intrinsically p-type semiconductors with large hole mobilities of 110 cm2 V−1 s−1,249,250 a similar magnitude to those found in MAPI single crystals (90 cm2 V−1 s−1).48 Furthermore, they are both solution processable which allows for the possibility of cheap and low cost device manufacturing through roll-to-roll printing.245 However, while GeSe has recently gained attention as a possible record breaking thermoelectric material,251 there are, to our knowledge, no reports of functioning germanium chalcogenide-based solar devices in the literature.
6 Antimony absorbers
Antimony compounds are used widely in therapeutics, and their manufacture carries little risk with modern regulations, and so present a further non-toxic alternative to lead.252 In addition to being more than twice as cheap per kg than tin,253 the 5s and 5p orbitals of antimony match well with those of p-block anions, often leading to a strong lone pair effect and potentially benevolent properties when in its 3+ oxidation state. However, this precludes direct integration into the AMX3 formula—instead, we review the wide variety of antimony compounds that have been studied for photovoltaics.
6.1 Antimony chalcogenides
The binary antimony chalcogenides have seen a great deal of research interest over the past 35 years, yet despite this interest, cell efficiencies remain below 10%. Both Sb2S3 and Sb2Se3 share a crystal structure consisting of 1D nanowires formed from Sb4Ch6 units, with the nanowires bound by weak Ch–Ch interactions. The Sb s2 lone pair is stereochemically active, forming a square pyramidal coordination around Sb, with the lone pair occupying space in-between the nanowires.254,255 This low dimensionality may be expected to reduce the overall functionality as an absorber material, however despite this, Sb2S3 and Sb2Se3 have both seen significant interest from the PV community.
Perhaps due to its simplicity, antimony sulfide has had a long, storied history as a photovoltaic absorber: thin films of Sb2S3 were examined photoelectrochemically in 1982 with PV applications in mind,256 however cells remained sparse until Savadogo and Mandal produced a series of n-Sb2S3 cells in the 1990's, ranging from a 3.9% efficient photoelectrochemical cell to 7.3% in a heterojunction with p-Ge and deposited with silicotungstic acid.257–259 Despite these prior successes, Sb2S3 only recently experienced a resurgence upon its use as a sensitizer with TiO2 and p-CuSCN as the hole transporting material (HTM), attaining efficiencies near 5%.260–263 Since, Sb2S3-sensitized cells have seen much work, as Seok and others have made recent strides in bringing efficiencies up to 7.5%264–267 in inorganic–organic heterojunction cells. This has largely been through the reduction of trap sites, however, there still remains a large Voc deficit limiting the growth of these cells. Measurements at low light have seen higher efficiencies of 8–9%, however the lack of comparable measurements with other materials makes this difficult to compare.268 With a high near-direct band gap of near 1.7 eV, and a high absorption coefficient,269,270 and multiple deposition techniques available,271,272 Sb2S3 remains a promising candidate material. First principles calculations have replicated the experimental band gap well, using hybrid DFT and meta-GGA functionals,273,274 and have identified that the valence electrons remained confined to the individual chains, but are diffuse within them: this dispersion indicates that the charge carriers should remain mobile.275,276
Despite the relative success of Sb2S3, within the last few years, there have been movements to develop the isostructural antimony selenide instead. Sb2Se3 has a lower band gap, around 1.1–1.3 eV in both nanostructures277 and thin films,278–280 than Sb2S3 and retains a high optical absorption coefficient of ∼105 cm−1.279–281 This high absorption may disguise a fundamental indirect band gap, which is only slightly lower in energy, as observed via DFT,282 quasiparticle band structure calculations,276 and UV-vis283 and so, like the sulfide, Sb2Se3 has been referred to as a ‘effectively direct band gap’ semiconductor. Additionally, theoretical band alignment with TiO2 indicates that with improvements in Voc, the lower band gap (and thus high possible Jsc) of Sb2Se3 compared to Sb2S3 could lead to higher attainable efficiencies in pnictogen-chalcogenide sensitised solar cells.284
Other than a <1% efficiency cell in 2002,278 almost all advances in Sb2Se3 cells began in 2014, with the publication of a TiO2-sensitized inorganic–organic cell of 3.2% from Choi et al.285 and a 1.9% thin film CdS/Sb2Se3 cell.286 Since then, Tang and co-workers have driven the development of the thin film architecture, reaching 3.7% by the end of 2014,287,288 and 5.6% in 2015.243,289 Additionally, they have indicated that vertically aligned growth of the 1D structure of Sb2Se3 means that high carrier transport can be maintained throughout the Sb2Se3 layer, while grain boundaries in the material will form parallel to the chains, making them inherently benign, reducing a major source of losses in polycrystalline cells (Fig. 6a).243 This applies to many other antimony and bismuth(III) materials, including Sb2S3 but also SbSI and the other members of the V–VI–VII series, where the stereochemically active lone pair enforces a 1-D topology—this presents a major advantage for the future development of these materials in photovoltaics.
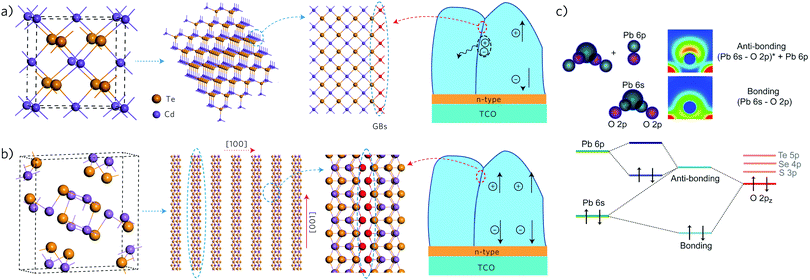 |
| Fig. 6 (a and b) The role of grain boundaries (GBs) as recombination centres in CdTe and Sb2Se3 solar cells. (a) The 3D structure of CdTe results in dangling bonds at GBs (illustrated by red rods), which act as defects that can cause unwanted recombination of charge carriers. (b) In contrast, the 1D ribbon structure of Sb2Se3, if oriented in the [001] direction, permits benign GBs due to saturation of the terminal atoms (red spheres). (a and b) Reprinted with permission from ref. 243. Copyright 2015, Nature Publishing Group. (c) Schematic of the orbital interactions that result in the formation of the stereochemical lone pair in PbO (top panel) and the corresponding molecular orbital diagram (bottom panel). Reprinted with permission from ref. 207. | |
In addition to the binary sulfides, a smaller section of work has examined the solid solution between the sulfide and selenide—with a tunable band gap between 1.3–1.7 eV,290 and high absorption in both binary systems, this presents an opportunity for tunable photovoltaic materials. Despite low initial efficiencies below 1%,291 recent work has used the advances in deposition techniques and the adoption of poly(3-hexylthiophene) (P3HT) as an efficient hole transporting material to attain an efficiency of 6.6%, close to the most successful Sb2S3 cells.292
6.2 Antimony chalcohalides
The ternary antimony chalcohalides adopt a 1D structure very similar to that of the simple chalcogenides mentioned above: the antimony atoms occupy a pseudo-square pyramidal configuration with sulfur and iodine in a dimeric unit that infinitely repeats along a, with the stereochemically active lone pair occupying the final position in the Sb octahedron.293 What distinguishes SbSI from the binary chalcogenides, however, is its photoferroicity: SbSI undergoes a ferroelectric phase transition,294 giving a phase with spontaneous polarization, allowing for anomalous photovoltaic effects beyond that of usual semiconductors, with the potential for very high Voc. Walsh and co-workers discuss this in their 2015 work,54 also calculating its electronic structure—like Sb2S3, it possesses a slightly indirect band gap of around 1.9 eV, in concordance with experiment,295 but also low effective masses. Despite this, as the authors highlight, SbSI and SbSeI have so far been passed over for other materials. In 2016, Walsh and co-workers revisited the V–VI–VII compounds, including SbSBr, using quasiparticle GW theory, obtaining mostly similar band gaps in addition to band alignments—this should allow for semiconductor contacts to be tailored for these materials.296
6.3 Copper antimony chalcogenides
In contrast, the ternary copper antimony chalcogenides have seen a great deal of interest in photovoltaic applications. CuSbS2 has seen perhaps the most attention—Nair and co-workers investigated p-type CuSbS2 in combination with intrinsic Sb2S3 to create a p–i–n device in 2005,297 however the early stage of development meant both the Jsc and Voc were relatively low. Fundamentally, however, CuSbS2 displays suitable electronic properties, with a band gap measured around 1.5 eV298 and good carrier mobilities of 20 to 49 cm2 V−1 s−1, although high conductivity has been found to be strongly dependent on the precise composition.253,299 Additionally, synthesis has been possible via a number of deposition and chemical routes.300–302 Its crystal structure, together with CuSbSe2, is related to the 3D chalcopyrite structure of the successful photoabsorber CuInSe2, however the greater stereochemical effect of the Sb s2 lone pair causes a distortion, resulting in a layered structure (Fig. 7a).303 Walsh et al., using DFT calculations, have discussed the electronic and structural effect of the lone pair in these materials: in concordance with the revised lone pair effect model for post-transition metal compounds,207 the antimony s states mix with the sulfur p states to allow further mixing with antimony p states, leading to antibonding states close to the top of the valence band and the structural distortion (Fig. 6b). This study also finds the band gaps to be indirect, although like the binary chalcogenides, only by 0.1–0.2 eV. This has been supported by subsequent theoretical work,304,305 which have additionally evaluated high absorption coefficients (>105 cm−1). Yu et al. corroborated these results, and used them to indicate that CuSbS2 and CuSbSe2, by possessing relatively flat band edges with a high Density of States there, will give stronger absorption than the well-known absorber CuInSe2.57 Using their metric of spectroscopically limited maximum efficiency (SLME), which improves upon the Shockley–Queisser limit by including non-radiative effects and film absorption, the SLMEs of CuSbS2 and CuSbSe2 were 23% and 27% respectively, comparable to or higher than that of CuInSe2. Cell efficiencies have, so far, have been unable to reach this level: CuSbS2 has improved significantly from that initial cell with multiple cells, either with CdS or sensitizing TiO2, now exceeding 3%,306–308 and CuSbSe2 has matched this, with cells of 1.3% and 3.5%309,310 published so far. However, recently two strategies have seen improvements in CuSbS2 cells: annealing in Sb2S3 vapour followed by KOH etching was seen to almost double Jsc, Voc and fill factors, while using a co-evaporation fabrication method lead to the highest recorded open-circuit voltage of 526 meV.311,312 With the suitable optical and electronic properties seen above, and Yang et al. finding few deep defect levels to act as recombination centres,253 further growth in this area may well be possible (Fig. 7b).
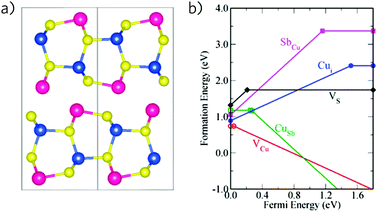 |
| Fig. 7 (a) Crystal structure and (b) intrinsic defect formation energies of CuSbS2. Adapted with permission from ref. 253. Copyright 2014, American Chemical Society. | |
6.4 Cesium and hybrid antimony compounds
Perhaps the most relevant antimony compounds to the hybrid perovskite family, however, are the cesium antimony halides and the cesium/methylammonium antimony sulfides. The 2D P
m1 phase of Cs3Sb2I9 was recently the subject of study by Saparov et al.;313 it occupies a “defect perovskite” structure, where
of the antimony sites of a hypothetical ‘Cs3Sb3I9’ perovskite unit are vacant, creating layers of corner-sharing Sb–I octahedra with a small inter-layer separation. This structure was found theoretically and experimentally to have an indirect 2.05 eV band gap, with carrier effective masses close to 0.5m0 and a high absorption coefficient. However, their prepared thin film cells displayed a Voc of ∼0.3 eV and low efficiencies, theorised to be a result of multiple deep defect levels effecting facile non-radiative recombination. Study of the methylammonium hybrid antimony iodide, (CH3NH3)3Sb2I9, has been primarily limited to spectroscopic studies on the dynamics of the methylammonium cation,314,315 however 2016 has seen the first production of a cell by Hebig et al., demonstrating an efficiency of 0.5%, improving upon that of the cesium compound.316 The cell demonstrated a much higher Voc of 0.90 eV, however a low Jsc of 1.0 mA cm−2 plus an Urbach tail energy of 62 meV indicating significant disorder in the amorphous absorber layer, suggests optimization of synthesis and cell construction will be required to produce viable cells.
Beyond this, work on hybrid antimony iodides remains limited: while some lower dimensionality perovskites have been reported, electronic characterisation is scarce.317,318 The antimony sulfides Cs3Sb8S13 and (MA)2Sb8S13 have also come to attention recently in the work of Yang et al.,273 who examined these two compounds with hybrid DFT. Both compounds demonstrate significant similarities with the parent Sb2S3 phase, such as the stereochemically active lone pair, similar, if higher band gaps, and a multi-valley band structure—which should lead to low radiative recombination, while retaining a high optical absorption.
7 Bismuth absorbers
Bismuth is particularly notable as a heavy metal as, unlike its neighbours of lead, polonium and thallium, it demonstrates very little evidence of toxicity.319,320 As such, it has seen increasing use in catalysis and organic synthesis as ‘green chemistry’ becomes ever more crucial;321,322 the rise of the lead halide perovskites in the perovskite community has lead to some considering whether bismuth compounds can reach a similar level of success.129
7.1 Bismuth sulfide
Bismuth sulfide, like the binary antimony chalcogenides, has had a long research history within the solar community, with the first thin films and photoelectrochemical cells being developed in the 1980's.323,324 Most research on bismuth selenide has focused on its rhombohedral topological insulator phase,325 as the 1.25 eV orthorhombic phase has only recently been isolated in a single-phase.326 The use of chemical deposition techniques to produce bismuth sulfide has seen considerable interest since,327–329 as offering a low temperature, facile synthesis is highly desirable for device manufacture, although the quality and crystallinity of films may suffer compared to evaporation techniques.330,331 Nevertheless, chemically deposited thin films of Bi2S3 have been used in combination with lead chalcogenides to produce cells of 0.5% efficiency in 2011,332 and 2.5% in 2013.333 With an optical band gap measured between 1.3–1.6 eV experimentally332,334 and through GW calculations,276 a high absorption coefficient, and low toxicity elements, Bi2S3 is a promising material for PV (Fig. 8a). Bi2S3 is intrinsically n-type,335 and hence has been particularly successful in heterojunctions with traditional p-type materials, such as crystalline silicon,336 or PbS quantum dots; the latter devices have neared 5% efficiency with the ‘bulk-nano’ heterojunction architecture leading to much longer carrier lifetimes and quadrupled Jsc in comparison with a bilayer architecture.337,338 Recently, two areas which have seen particular success are its use as a TiO2 sensitizer, reaching 2.5% efficiency in 2015,339,340 and in nanostructures.341–343 In particular, hybrid solar cells containing nanocrystalline Bi2S3 in combination with the organic absorber P3HT have seen great progress from the group of Konstantatos and others, with cell efficiencies rising from below 1% in 2011 to 3.3% in 2015,344–346 and the exploration of size-dependent tunability and passivation of surface defects.347,348
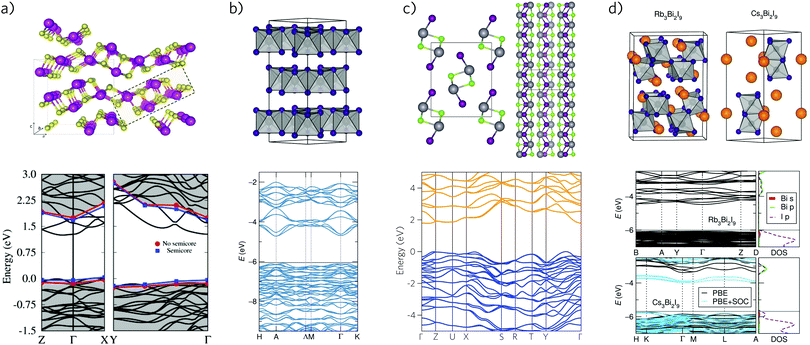 |
| Fig. 8 Crystal structure and band structure of bismuth based solar absorbers: (a) Bi2S3—adapted with permission from ref. 335. Copyright 2016, American Chemical Society; and ref. 276. Copyright 2013, American Physical Society (b) BiI3—adapted with permission from ref. 349. Copyright 2015, American Chemical Society; and ref. 350. Copyright 2015, AIP Publishing LLC. (c) BiSI—adapted with permission from ref. 351. (d) Rb3Bi2I9 and Cs3Bi2I9—adapted with permission from ref. 349. Copyright 2015, American Chemical Society. | |
7.2 Bismuth iodide
Like the antimony and bismuth chalcogenides and chalcohalides, bismuth iodide, BiI3, was recently highlighted by Brandt et al. as a potential solar absorber material due to its suitable band gap and the likelihood of good defect tolerance.37 Prior to this, its involvement in photovoltaics has been limited—most research has focused on its use as a radiation detector,352,353 with a single report of its use as a hole-transport layer in organic solar cells, where its performance was observed to be comparable to PEDOT:PSS.354 Occupying a layered rhombohedral crystal structure,355 its band gap was the subject of considerable variance, with values ranging from 1.99 eV with ellipsometry to 1.43 eV with DFT calculations (Fig. 8b).356,357 Podraza et al. were able to rationalise this through the low optical absorption obscuring the actual indirect band gap of 1.67 eV, in close agreement with other calculations.358,359 With a suitable band gap for PV applications, it has thus been the subject of study by Seshadri and co-workers, producing a cell with 0.3% efficiency; the Voc of this cell was noted to be low due to the poor alignment of BiI3 with the hole and electron transporting layers.349,350 Brandt et al. have also further studied this compound, finding a much higher absorption coefficient than previously recorded, however photoluminescence revealed an estimated, short carrier lifetime of 180–240 ps which, in combination with a high resistivity of ∼108 Ω cm−1, is likely to severely hinder conductivity.360 Recent computational study of BiI3 monolayers, however, predicted them to be stable, and show significantly improved IR-absorbance in a bilayer with graphene, raising the possibility of photoactive heterostructures.361
7.3 Bismuth chalcohalides
With analogous 1D crystal structures to their antimony counterparts,362–364 together with suitable, slightly indirect band gaps of 1.59 eV and 1.29 eV respectively,365 it is understandable that BiSI and BiSeI should also be considered for photovoltaic applications (Fig. 8c). However, despite interest in its photoconduction and photovoltaic behaviour in the 1960's,366,367 it is only very recently that the bismuth chalcoiodides have been thoroughly assessed in this manner. In 2012, Mullins and co-workers published two studies on this topic—the first of these was a thorough characterization study of BiSI thin films, finding a high absorption coefficient (5 × 104 cm−1), photocurrents of up to 5 mA cm−2 under AM1.5G illumination and a maximum Voc of 370 mV with a I−/I3− couple.368 The second examined the effect of selenium doping, allowing for a decrease in band gap of 0.15 eV, and attempted both photoelectrochemical and solid-state cells, with 0.25% and 0.01% efficiency respectively,369 however BiSI was observed to degrade under electrochemical conditions. Recent theoretical work has explained the poor solid-state behaviour: the conduction band alignment of BiSI means that the choice of p-CuSCN as the hole transporting material limits Voc considerably, and there will be poor electron transfer to the FTO electrode, as used by Mullins and co-workers.351 As such, there should be great potential for improvement—with antibonding states at the valence band maximum, and a high static dielectric constant recently evaluated,370 BiSI films should possess the high degree of defect tolerance identified by Brandt et al. as a highly desirable PV property.37
While the bismuth oxyhalide family (BiOX, X = Cl, Br, I) have been investigated for a variety of applications, most notably as photocatalysts for organic pollutant decomposition or water splitting,371–374 a small number of reports have examined BiOI for photovoltaic applications. BiOI has the lowest band gap of the family, measured between 1.6 eV and 2.0 eV,372,375,376 meaning that while it demonstrates the weakest photoelectrochemical behaviour,377 it is also likely the best candidate for photovoltaics. The first attempt at a cell in 2009, using a iodide couple in ethylene glycol attained a moderate Voc of 461 mV, but a very low short circuit current (20.4 μA cm−2), likely due to the poor conduction between the BiOI and its chitosan matrix.378 Directly coating the FTO electrode with BiOI was observed to increase Jsc by a factor of 10,379 yet the most significant improvement was seen recently with a similar cell architecture, with a Jsc of 3.8 mA cm−2 and an efficiency of 1.03%.380 Interestingly, BiOI has also seen use recently in combination with Bi2S3 as the absorber layer—while short-circuit currents were again above 1 mA cm−2, low fill factors meant efficiencies were low.381 Attempts to utilise BiOCl and BiOBr in other DSSCs have also met with low currents and efficiencies.382
7.4 Noble metal bismuth chalcogenides and halides
CuBiS2, like the copper antimony chalcogenides, has seen substantial theoretical interest within the last four years,40,304,383–386 with much attention paid to the structural effect of the bismuth lone pair, and the high absorption coefficient (∼105 cm−1)—the band gaps obtained (1.5–1.6 eV) also correlate well with experimental measurements (1.6–1.8 eV).387,388 Additionally, CuBiS2 has been shown to demonstrate moderate hole mobilities of >10 cm2 V−1 s−1,389,390 and although previously assigned as an n-type conductor,387,388 recent theoretical and experimental work strongly suggests it is p-type.385,390 Despite this substantial effort towards characterisation, however, detailed study of defects and production of a cell remains an open challenge.
Alternate copper bismuth sulfides, on the other hand, have seen a little more success. Interest in Cu3BiS3 from the photovoltaic community mostly began in the 2000's: Estrella et al. characterised thin films of Cu3BiS3 in 2003, finding a band gap within 1–1.6 eV, a high optical absorption coefficient (>105 cm−1) and a photocurrent response;391 Gerein and Haber were able to replicate the band gap (1.3 eV) and absorption coefficient, in addition to finding low resistivity in high quality annealed films.392 Since then, experimental studies have examined its photovoltaic behaviour, finding that surface trap states may be passivated in a heterojunction with In2Se3,393,394 and its excitonic behaviour through Raman and Photoluminescence measurements.395 Theoretical results have evaluated the fundamental indirect band gap at around 1.7 eV, but have also reproduced the high absorption coefficient in addition to predicting a relatively low hole effective mass (<0.5m0) and the efficiency improvement on O-doping.396–398 Most recently, a photoelectrochemical energy conversion efficiency of 1.28% was obtained in a Cu3BiS3-sensitized TiO2 cell, giving a strong indication of the potential of these materials.399 Cu4Bi4S9 has also seen particular success as a sensitizer to In2Se3, obtaining cells with efficiencies between 3.9% and 6.2% with a variety of oxide contacts, with the Cu4Bi4S9 addition noted to particularly improve the surface photovoltages.400
Most notably, however, AgBiS2 has seen a massive stride in photovoltaic efficiency very recently, much more so than its copper analogues. Also unlike CuBiS2, nanocrystalline- and high temperature bulk-AgBiS2 occupies a 3D cubic disordered rocksalt structure,401,402 which has seen previous interest due to its high temperature order–disorder transition and its effect on thermoelectric behaviour.403,404 While the bulk has been recorded to have an optical band gap of 0.9 eV,405 strong quantum confinement effects have meant that quantum dot thin-films have band gaps reported at 1.0–1.3 eV, although with one study reporting 2.67 eV; that study also reports a very strong absorption coefficient above 105 for low frequencies.405–407 Despite this, initial AgBiS2 QD-sensitized TiO2 cells reported low efficiencies of 0.53% and 0.95%, both of which suffered from low fill factors and low current densities,402,408 while AgBiS2 QDs have also been seen to improve on Pt as a contact material.409 Recent work by Bernechea et al. however, using tetramethylammonium iodide-treated AgBiS2 nanocrystals in a p–i–n junction between PTB7 and ZnO, has recently given a record cell of 6.3% efficiency, equaling many of the record antimony and bismuth-based cells, with a high Jsc of 22 mA cm−2 and FF of 0.63.410 A relatively low Voc of 0.45 eV was attributed to trap-assisted recombination, and so improvements in synthesis could easily lead to significantly easier charge transport and even higher efficiencies in the future.
Also notable is the recent production of a cell based on cubic AgBi2I7, a compound that has only been sparsely characterised previously.411 Sargent and co-workers investigated the AgI–BiI3 solid solution, producing a thin film of AgBi2I7, and reporting a direct band gap of 1.86 eV.412 A subsequent nanocomposite cell, with AgBi2I7 between mesoporous TiO2 and P3HT, gave an efficiency of 1.12%, with a high fill factor of 0.67, but limited by relatively lower current density and voltage. As with many of the above compounds, further attention could lead to significant improvements in the future.
7.5 Cesium and hybrid bismuth iodides
The cesium bismuth iodide Cs3Bi2I9 and its methylammonium counterpart, (CH3NH3)3Bi2I9, have come under attention similar to the antimony analogue. While past studies have performed detailed study concerning the low temperature (∼200 K) ferroelastic phase transition of Cs3Bi2I9,413–415 and the vibrational properties of (CH3NH3)3Bi2I9,416 thorough electronic characterization of the room temperature phases has only been performed recently. This structural behaviour may still be relevant, however, given that exciton–phonon coupling in the bromide analogue, Cs3Bi2Br9, has been shown to lead to a much stronger exciton binding energy than the lead halide perovskites.417 Unlike the layered Cs3Sb2I9, the bismuth compounds occupy the ‘zero dimensional’ Cs3Cr2Cl9 structure, with isolated [Bi2I9]3− dimers of face-sharing octahedra surrounded by the free Cs+ or (CH3NH3)+ cations,418–420 raising the possibility of lower carrier mobilities. Indeed, Lehner et al.'s theoretical and experimental characterisation of Cs3Bi2I9 found much lower band dispersion in comparison with MAPI, indicative of low mobilities, together with a relatively high indirect optical band gap of 1.9 eV (Fig. 8d).349 Nevertheless, Cs3Bi2I9 was used as an absorber on mesoporous TiO2 by Park et al. to give a best cell with 1% efficiency and a Voc of 0.85 eV,421 a significant improvement on the equivalent (CH3NH3)3Bi2I9 cells which had efficiencies below 0.2% and much lower Voc and Jsc. Other attempts to use (CH3NH3)3Bi2I9 since, with alternate organic hole transporting layers, have seen small improvements in Jsc422,423 and fill factor,424 but efficiencies remain low, despite a similar band gap of 2.1 eV and strong absorption.425,426 Buonassisi and co-workers also studied the photoluminescence behaviour of (CH3NH3)3Bi2I9, finding a decay time of 760 ps—up to 103 lower than the highest recombination lifetime in MAPI films, however it is noted that a <1 ns lifetime is comparable to the initial values recorded for popular absorbers such as Cu2ZnSnSe4 and SnS.425 A low PL quantum efficiency of 0.4% was also recorded and attributed to non-radiative recombination pathways; Park et al. found defect states in the XPS of Cs3Bi2I9 that suggest it may be similarly limited.421 It is evident that the photovoltaic behaviour of both materials may be enhanced if further work to reduce the impact of these pathways is performed, and, as both studies also found that the bismuth iodides were stable in air over a month, these compounds do present a potential avenue for hybrid bismuth PV materials.
(CH3NH3)3Bi2I9 is not the only inorganic–organic bismuth iodide to have been examined for photovoltaic activity, with the one-dimensional hexanediammonium bismuth iodide, (H3NC6H12NH3)BiI5, recently trialled in a mesoporous TiO2 architecture. Like the bismuth iodides above, these devices also possessed low Voc and Jsc, and a band gap close to 2 eV, however they also showed greater film coverage and thermal stability than MAPI.429
7.6 Double perovskites (elpasolites)
Very recently, there have been significant efforts to move beyond the AIMIIX3 perovskite formula to the double perovskite AI2MIMIIIX6. Crucially, this allows the expansion of the perovskite motif beyond Group 14 ions on the M site while retaining the successful 3D perovskite structure (Fig. 9a). While double perovskites in the oxide family are well known as fuel cells,433,434 only a few relevant halides such as Cs2NaBiCl6, with absorption much higher in energy than the ideal photovoltaic range, are known.435,436 In 2015, however, Giorgi and Yamashita proposed a simple substitution of Tl+ and Bi3+, in place of Pb2+, into the tetragonal MAPI structure using DFT, finding a theoretical bandgap of 1.68 eV, compared with MAPI's 1.62 eV using the PBE functional.437 However, as the authors admit, Tl presents an environmental risk and its possible replacement by its neighbour InI would likely lead to instability issues, similar to SnII.
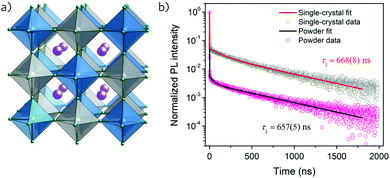 |
| Fig. 9 (a) Crystal structure of Cs2BiAgBr6. Adapted with permission from ref. 427. Copyright 2015, American Chemical Society. (b) Time-resolved room temperature photoluminescence (PL) of single-crystal and powder Cs2BiAgBr6, including fits for the PL decay time (τ). Reprinted with permission from ref. 428. Copyright 2016, American Chemical Society. | |
Instead, at the beginning of 2016, multiple separate groups have published on the Cs2AgBiX6 family. Slavney et al. synthesised Cs2AgBiBr6 and discuss its photoluminescence behaviour, finding a long decay time of 660 ns which is attributed to a long recombination lifetime dominated by non-radiative recombination, similar to that found in MAPI, and estimating the indirect band gap at 1.95 eV (Fig. 9b).428 McClure et al. were able to synthesise both the bromide and chloride, finding indirect bandgaps of 2.19 eV and 2.77 eV respectively from diffuse reflectance spectroscopy and supporting these observations with DFT calculations, finding hole effective masses slightly lower than those in the analogous methylammonium lead bromide.452 Additionally, both studies examined the stability of these materials: Slavney et al. found the bromide stable up to 430 °C and no evidence of degradation under either moisture or light after 30 days, however, while McClure et al. found no significant decomposition in the chloride, the reflectance and XRD pattern of Cs2AgBiBr6 was observed to degrade when stored in light and air. Volonakis et al. have performed a wider theoretical screening of the double perovskites using hybrid DFT, examining MI = Cu, Ag, Au and MIII = Bi, Sb and additionally synthesising Cs2BiAgCl6 to support their observations.427 Of those predicted, a linear decrease is observed in all cases down the halide group, while, for MI, the Ag compounds have higher band gaps, and Au the lowest; as such, Cs2AgSbI6, Cs2BiCuI6 and Cs2BiAuBr6 are those observed to have band gaps within the optimal 1.0–1.5 eV range. Further work from the Giustino group has seen the accurate prediction of the band gaps of Cs2BiAgCl6 and Cs2AgBiBr6 using quasiparticle GW theory.453 Recent work by the Cheetham and co-workers has seen the synthesis of the methylammonium double perovskites (MA)2KBiCl6 and (MA)2TlBiBr6: however, (MA)2KBiCl6's indirect band gap of 3.04 eV is too high for efficient use in photovoltaics, and while the 2.16 eV bandgap of (MA)2TlBiBr6 is direct, the inclusion of toxic thallium, as discussed above, would minimize the benefit of removing lead.454,455 It is clear that while the double perovskite motif allows the replacement of Pb, their wide, indirect band gaps and the potential issue of stability may be problematic for further PV applications.
This split-ion approach for producing bismuth double-perovskites has similarly been proposed on the anion site, with a mix of chalcogenides and halides: DFT calculations predicted the hypothetical CH3NH3BiSI2 to have a similar band gap, dielectric behaviour and effective masses to MAPI.456 However, another wide-ranging theoretical study on the analogous inorganic AM(ChIIXI)3 (A = Cs, Ba, M = Sb, Bi, Ch = O, S, Se, Te and X = F, Cl, Br, I) compounds has found that all were unstable to decomposition, and the authors' attempts to synthesise these compounds found no evidence that the target phases were produced.28 As Hong et al. reflect, it is clear that when such alternatives, particularly quaternary compounds with a larger number of competing phases, are predicted, assessments of their stability should not be neglected.
7.7 Further inorganic–organic bismuth compounds
Further to those previously mentioned, there exists a massive library of extant hybrid inorganic–organic bismuth and antimony compounds due to the surge in interest in the 1990's and early 2000's. Several reduced dimensionality bismuth iodides exist, yet are relatively under-characterised: these include guanidinium, iodoformamidinium and a variety of alkyl and photoactive-cation bismuth iodides, many of which were synthesised by David Mitzi and his co-workers.100,103,317,457–461 Indeed, there are still many new bismuth compounds being discovered even this year,462 some of which may already be promising PV candidate materials.463,464 Given the diversity of coordination, dimensionality and organic character in these examples alone, it is clear there already exists a wide parameter space of potential inorganic–organic bismuth compounds. A full survey of these materials is beyond the scope of this review, yet a number of excellent reviews cover this section of bismuth chemistry already, particularly low-dimensionality structures.71,465,466 Nevertheless, the field of hybrid-organic bismuth compounds would appear to open up an opportunity: a new frontier of non-toxic, optoelectronic materials waiting to be examined and explored by the photovoltaic community.
8 Outlook and conclusion
Table 1 gives the highest recorded efficiencies and device specifications for all ns2 solar absorbers discussed in this Review. Lead-based devices currently outperform all alternatives—this is, in part, due to the enormous research effort these materials have experienced but also to the excellent properties of lead, highlighted in our introduction. Despite the similarities in the electronic properties of the group 14 metals and the long-standing research interest in tin solar absorbers, tin-based devices still lag behind their lead counterparts. This can be attributed the sensitivity of Sn2+ (and similarly, Ge2+) to oxidation, which hampers device fabrication and leads to efficiency losses. It is therefore essential that alternative schemes to stabilise tin-based devices are developed. Antimony devices have seen persistent improvements to their efficiencies since 2009 and show the second highest performance of any material behind lead. Their earth-abundant composition make them attractive as emerging solar absorbers and with increased attention, efficiencies should continue to rise. Analogously, there has been an increase in reports of bismuth-based devices over the last few years, spurred on by its non-toxic nature and ideal electronic properties, which are expected to be ideal for solar cell applications. As can be seen in Fig. 10, bismuth containing absorbers have recently begun to show efficiencies comparable with the best antimony and tin alternatives.
Table 1 Highest recorded efficiencies for a range of ns2 solar absorbers, with corresponding device open-circuit voltage (Voc), short-circuit current (Jsc), fill factor (FF), band gap (Eg), and fabrication details. Nanocomposite devices are those containing mesoporous TiO2 (m-TiO2) impregnated with absorber material. SILAR and ALD refer to the successive ionic layer adsorption and reaction, and atomic layer deposition processes, respectively
|
Absorber |
PCE (%) |
V
oc (V) |
J
sc (mA cm−2) |
FF (%) |
E
g (eV) |
Architecture |
Cell type |
Deposition method |
Ref. |
The highest recorded efficiency for a hybrid halide lead perovskite solar cell is 22.1%, however, only limited technical specifications for this device have been released.467 As such, we report details for the next highest efficiency device here.
|
Pb
|
CH3NH3PbI3 |
19.1 |
1.09 |
22.4 |
80 |
1.55 |
FTO/c-TiO2/mTiO2/perovskite/spiro-OMeTAD/Au |
Nanocomposite |
Spin coating |
430
|
(MA,FA,Cs)PbI3 |
21.1a |
1.15 |
23.5 |
78 |
1.55 |
FTO/c-TiO2/mTiO2/perovskite/spiro-OMeTAD/Au |
Nanocomposite |
Spin coating |
431
|
CH(NH2)2PbI3 |
20.1 |
1.06 |
24.7 |
78 |
1.48 |
FTO/NiO/perovskite/PCBM/LiF/Al |
Planar heterojunction |
Spin coating |
432
|
CsPbI3 |
2.9 |
0.80 |
12.0 |
— |
1.73 |
FTO/c-TiO2/perovskite/spiro-OMeTAD |
Planar heterojunction |
Spin coating |
80
|
CsPbI2Br |
9.8 |
1.10 |
11.89 |
75 |
1.92 |
FTO/c-TiO2/perovskite/spiro-OMeTAD/Ag |
Planar heterojunction |
Spin coating |
79
|
FA0.83Cs0.17Pb(I0.6Br0.4)3 |
17.9 |
1.19 |
19.4 |
79 |
1.74 |
FTO/SnO2/PCBM/perovskite/spiro-OMeTAD/Ag |
Planar heterojunction |
Spin coating |
89
|
(PEA)2(MA)59Pb60I181 |
15.4 |
1.09 |
19.1 |
74 |
1.6 |
FTO/c-TiO2/perovskite/spiro-OMeTAD/Au |
Planar heterojunction |
Spin coating |
110
|
(CH3NH3)2Pb(SCN)2I2 |
8.3 |
0.87 |
15.1 |
63 |
1.5–2.1 |
FTO/c-TiO2/perovskite/spiro-OMeTAD/Au |
Planar heterojunction |
Spin coating |
120
|
PbS |
9.2 |
0.54 |
30.0 |
58 |
11.0 |
FTO/TiO2/PbS/MoO3/Au/Ag |
Quantum dot |
Spin coating |
148
|
PbSe |
6.2 |
0.52 |
23.4 |
52 |
— |
ITO/TiO2/PbSe/MoO3/Al |
Quantum dot |
Spin coating |
157
|
|
Sn
|
CH3NH3SnI3 |
6.4 |
0.88 |
16.8 |
42 |
1.2 |
FTO/c-TiO2/mp-TiO2/perovskite/spiro-OMeTAD/Au |
Nanocomposite |
Spin coating |
173
|
CH(NH2)2SnI3 |
4.8 |
0.32 |
23.7 |
63 |
1.4 |
FTO/c-TiO2/mp-TiO2/perovskite/spiro-OMeTAD/Au |
Nanocomposite |
Spin coating |
164
|
CsSnI3 |
2.0 |
0.24 |
22.7 |
37 |
1.3 |
FTO/c-TiO2/mp-TiO2/perovskite/m-MTDATA/Au |
Nanocomposite |
Spin coating |
181
|
SnS |
4.4 |
0.37 |
20.2 |
58 |
1.1 |
ITO/ZnO/Zn(O,S):N/SnO2/SnS/Mo |
Planar heterojunction |
ALD |
193
|
|
Ge
|
CsGeI3 |
3.2 |
0.53 |
10.5 |
53 |
1.6 |
FTO/TiO2/perovskite/P3HT/Au |
Planar heterojunction |
Spin coating |
239
|
CH3NH3GeI3 |
0.2 |
0.15 |
4.0 |
30 |
11.9 |
FTO/c-TiO2/mp-TiO2/perovskite/spiro-OMeTAD/Au |
Nanocomposite |
Spin coating |
238
|
|
Sb
|
Sb2S3 |
7.5 |
0.71 |
16.1 |
65 |
1.7 |
FTO/TiO2/mp-TiO2/Sb2S3/PCPDTBT/PEDOT:PSS/Au |
Nanocomposite |
Spin coating |
266
|
Sb2Se3 |
5.6 |
0.40 |
25.1 |
57 |
1.1–1.3 |
FTO/CdS/Sb2Se3/Au |
Planar heterojunction |
Spin coating |
243
|
Sb2S3−xSex |
6.6 |
0.48 |
24.9 |
56 |
1.3–1.7 |
FTO/TiO2/mp-TiO2/absorber/P3HT/Au |
Nanocomposite |
Spin coating |
292
|
CuSbS2 |
3.2 |
0.47 |
15.6 |
44 |
1.6 |
Al/n-ZnO/i-ZnO/CdS/CuSbS2/Mo |
Planar heterojunction |
Spin coating |
308
|
CuSbSe2 |
3.5 |
0.35 |
22.8 |
— |
1.1–1.3 |
MgF/Ni:Al/n-ZnO/i-ZnO/CdS/CuSbSe2/Mo |
Planar heterojunction |
DC sputtering |
310
|
(MA)3Sb2I9 |
0.5 |
0.89 |
1.0 |
55 |
2.1 |
ITO/PEDOT:PSS/(MA)3Sb2I9/PC61BM/nano-ZnO/Al |
Planar heterojunction |
Spin coating |
316
|
Cs3Sb2I9 |
<1.0 |
0.31 |
— |
— |
2.1 |
FTO/c-TiO2/Cs3Sb2I9/PTAA/Au |
Planar heterojunction |
Co-evaporation |
313
|
|
Bi
|
Bi2S3 |
2.5 |
0.50 |
9.3 |
54 |
1.3–1.6 |
FTO/TiO2/CdS/Bi2S3/ZnS |
Quantum dot |
SILAR |
333
|
Bi2S3/P3HT |
3.3 |
0.69 |
10.7 |
45 |
1.2 |
ITO/TiO2/Bi2S3:P3HT/MoO3/Au |
Planar heterojunction |
Spin coating |
346
|
Bi2S3/PbS QD |
4.9 |
0.40 |
24.2 |
50 |
1.2 |
ITO/PbS QD/PbS/Bi2S3/Ag |
Bulk nano-heterojunction |
Spin coating |
338
|
BiI3 |
0.3 |
0.42 |
1.7 |
45 |
1.8 |
FTO/TiO2/BiI3/PTAA/Au |
Planar heterojunction |
Spin coating |
350
|
BiSI |
<0.1 |
0.39 |
0.1 |
40 |
1.6 |
FTO/BiSI/p-CuSCN/FTO/Pt |
Planar heterojunction |
Spray pyrolysis |
369
|
BiOI |
1.0 |
0.61 |
3.8 |
45 |
1.8 |
FTO/TiO2/BiOI/(I3−/I−)/FTO/Pt |
Liquid electrolyte |
SILAR |
380
|
AgBiS2 |
6.3 |
0.45 |
22.1 |
63 |
1.3 |
ITO/ZnO/AgBiS2/PTB7/Ag |
Planar heterojunction |
Hot injection |
410
|
AgBi2I7 |
1.2 |
0.56 |
3.3 |
67 |
1.9 |
FTO/c-TiO2/mp-TiO2/AgBi2I7/P3HT/Au |
Nanocomposite |
Spin coating |
412
|
Cs3Bi2I9 |
1.1 |
0.85 |
2.2 |
60 |
2.2 |
FTO/TiO2/mp-TiO2/perovskite/spiro-OMeTAD/Ag |
Planar heterojunction |
Spin coating |
421
|
(CH3NH3)3Bi2I9 |
0.1 |
0.68 |
0.5 |
33 |
2.1 |
FTO/TiO2/mp-TiO2/perovskite/spiro-OMeTAD/Ag |
Planar heterojunction |
Spin coating |
421
|
(H3NC6H12NH3)BiI5 |
<0.1 |
0.40 |
0.1 |
43 |
2.1 |
FTO/c-TiO2/mp-TiO2/absorber/spiroOMeTAD/Au |
Nanocomposite |
Spin coating |
429
|
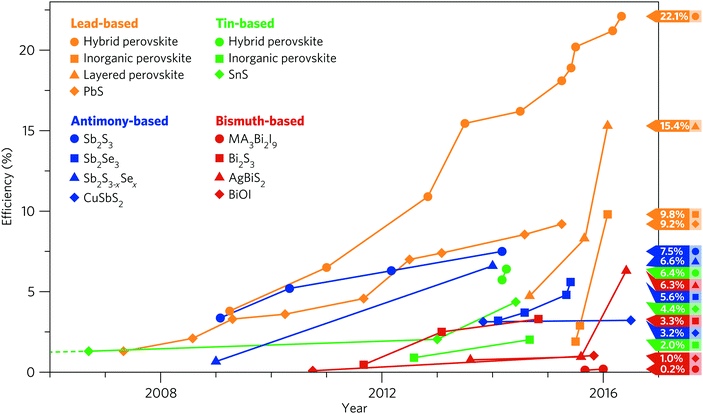 |
| Fig. 10 Efficiency improvements over time for a range of ns2 solar absorbers. Only materials with greater than one recorded device efficiency improvement are included. Devices with efficiencies lower than 0.1% have been omitted. Lead-based absorbers (orange): hybrid perovskite ref. 86, 184, 431, 432 and 438–442; inorganic perovskite ref. 79, 80, 443 and 444; layered perovskite ref. 96, 110 and 120; PbS ref. 147, 148 and 445–451. Tin-based absorbers (green): hybrid perovskite ref. 172 and 173; inorganic perovskite ref. 180 and 181; SnS ref. 192, 193, 200 and 224. Antimony-based absorbers (blue): Sb2S3ref. 260–262, 265, 266 and 278; Sb2Se3ref. 243, 278, 285, 288 and 289; Sb2S3−xSexref. 291 and 292; CuSbS2ref. 306 and 308. Bismuth-based absorbers (red): MA3Bi2I9ref. 421 and 422; Bi2S3ref. 333, 344 and 346; AgBiS2ref. 402, 408 and 410; BiOI ref. 379 and 380. | |
Several materials highlighted in this review show excellent promise as solar absorbers. The layered lead hybrid perovskites have seen a sharp rise in efficiency in recent months—comparable with the rise of MAPI—due to the development of a “quasi-2D” structure. This approach combines the beneficial increase in stability seen in the purely layered structures, with the excellent optical and electronic properties possessed by the 3D perovskites. In this way, stability and optical properties can be finely tuned to enable moisture tolerant devices with exceptional absorber characteristics. The bismuth containing material AgBiS2 has also seen rapid advances in efficiencies recently. Its 3D-connected cubic structure draws parallels with the hybrid perovskites and should allow for comparable charge transport properties.
The rise of the hybrid perovskites and emergence of MAPI has galvanised the photovoltaic community with a renewed focus. That an unknown and little studied material can overtake all other third-generation solar absorbers in such a short number of years firmly demonstrates the potential of an earth-abundant and cost-effective alternative to silicon technologies. Such a dramatic rise in efficiencies has relied on a multi-disciplinary approach and has brought together a decade's worth of advancements in the photonics, engineering and synthetic chemistry communities. Nonetheless, MAPI clearly possesses a fortuitous combination of properties that make it tolerant of a wide range of synthesis conditions and an ideal solar absorber. For this reason, the rise of MAPI should be seen as an outlier and not a precedent to be expected of all emerging absorbers. Instead, it is likely that the next generation of solar materials will require many years of development and optimisation before they can reach comparable efficiencies. Regardless, the emergent field of ns2 solar absorbers shows particular promise for the future of photovoltaic energy generation.
Acknowledgements
DOS acknowledges support from the SUPERSOLAR Solar Energy Hub (EP/J017361/1) for the provision of a flexible funding call award, EPSRC (EP/N01572X/1), and membership of the Materials Design Network. CNS is grateful to the Department of Chemistry at UCL for the provision of a DTA studentship, and AMG acknowledges Diamond Light Source for the co-sponsorship of a studentship on the EPSRC Centre for Doctoral Training in Molecular Modelling and Materials Science (EP/L015862/1). We would also like to acknowledge T. Gershon, A. Zakutayev, T. D. Veal, A. Walsh, R. Seshadri, R. G. Palgrave, T. Buonassisi, R. L. Z. Hoye, V. Steinmann, R. E. Brandt, P. K. Nair, B. C. Melot, S. Chen, D. B. Mitzi, T. K. Cheetham, H. J. Snaith, S. Lany, E. Arca, Y. Yan, H. Bronstein, J. D. Major, K. Durose, K. T. Butler, J. M. Frost, C. H. Hendon, J. M. Skelton, B. A. D. Williamson, A. J. Jackson, J. Buckeridge, S.-H. Wei, M. Van Schilfgaarde, C. Persson, F. Giustino, and P. M. Woodward for many illuminating discussions at conferences and the myriad of fascinating contributions they have published in this field.
References
- N. S. Lewis and D. G. Nocera, Proc. Natl. Acad. Sci. U. S. A., 2006, 103, 15729–15735 CrossRef CAS PubMed.
- W. A. Hermann, Energy, 2006, 31, 1685–1702 CrossRef CAS.
-
J. Nelson, The physics of solar cells, World Scientific, 2003, vol. 57 Search PubMed.
- J. Ondraczek, N. Komendantova and A. Patt, Renewable Energy, 2015, 75, 888–898 CrossRef.
- J. Peng, L. Lu and H. Yang, Renewable Sustainable Energy Rev., 2013, 19, 255–274 CrossRef CAS.
- W. Shockley and H. J. Queisser, J. Appl. Phys., 1961, 32, 510–519 CrossRef CAS.
- C. Wadia, A. P. Alivisatos and D. M. Kammen, Environ. Sci. Technol., 2009, 43, 2072–2077 CrossRef CAS PubMed.
- N. G. Dhere, Sol. Energy Mater. Sol. Cells, 2007, 91, 1376–1382 CrossRef CAS.
- M. A. Green, A. Ho-Baillie and H. J. Snaith, Nat. Photonics, 2014, 8, 506–514 CrossRef CAS.
- M. Grätzel, Nat. Mater., 2014, 13, 838–842 CrossRef PubMed.
- T. Baikie, Y. Fang, J. M. Kadro, M. Schreyer, F. Wei, S. G. Mhaisalkar, M. Graetzel and T. J. White, J. Mater. Chem. A, 2013, 1, 5628–5641 CAS.
- V. D'Innocenzo, G. Grancini, M. J. Alcocer, A. R. S. Kandada, S. D. Stranks, M. M. Lee, G. Lanzani, H. J. Snaith and A. Petrozza, Nat. Commun., 2014, 5, 3586 Search PubMed.
- A. Walsh, D. O. Scanlon, S. Chen, X. G. Gong and S.-H. Wei, Angew. Chem., 2015, 127, 1811–1814 CrossRef.
- Y. Deng, E. Peng, Y. Shao, Z. Xiao, Q. Dong and J. Huang, Energy Environ. Sci., 2015, 8, 1544–1550 CAS.
- G. Xing, N. Mathews, S. Sun, S. S. Lim, Y. M. Lam, M. Gratzel, S. Mhaisalkar and T. C. Sum, Science, 2013, 342, 344–347 CrossRef CAS PubMed.
- Y. Shao, Z. Xiao, C. Bi, Y. Yuan and J. Huang, Nat. Commun., 2014, 5, 5784 CrossRef CAS PubMed.
- C. Wehrenfennig, G. E. Eperon, M. B. Johnston, H. J. Snaith and L. M. Herz, Adv. Mater., 2014, 26, 1584–1589 CrossRef CAS PubMed.
- Y. Zhao and K. Zhu, J. Phys. Chem. Lett., 2013, 4, 2880–2884 CrossRef CAS.
- A. T. Barrows, A. J. Pearson, C. K. Kwak, A. D. F. Dunbar, A. R. Buckley and D. G. Lidzey, Energy Environ. Sci., 2014, 7, 2944 CAS.
- D. S. Bhachu, D. O. Scanlon, E. J. Saban, H. Bronstein, I. P. Parkin, C. J. Carmalt and R. G. Palgrave, J. Mater. Chem. A, 2015, 3, 9071–9073 CAS.
- G. Hodes and D. Cahen, Nat. Photonics, 2014, 8, 87–88 CrossRef CAS.
- J. A. Christians, J. S. Manser and P. V. Kamat, J. Phys. Chem. Lett., 2015, 6, 852–857 CrossRef CAS PubMed.
- G. Nagabhushana, R. Shivaramaiah and A. Navrotsky, Proc. Natl. Acad. Sci. U. S. A., 2016, 113, 7717–7721 CrossRef CAS PubMed.
- S. Guarnera, A. Abate, W. Zhang, J. M. Foster, G. Richardson, A. Petrozza and H. J. Snaith, J. Phys. Chem. Lett., 2015, 6, 432–437 CrossRef CAS PubMed.
- G. Niu, X. Guo and L. Wang, J. Mater. Chem. A, 2015, 3, 8970–8980 CAS.
- J. Yang and T. L. Kelly, Inorg. Chem., 2016 DOI:acs.inorgchem.6b01307.
- T. J. Jacobsson, J.-P. Correa-Baena, M. Pazoki, M. Saliba, K. Schenk, M. Grätzel and A. Hagfeldt, Energy Environ. Sci., 2016, 9, 1706–1724 Search PubMed.
- F. Hong, B. Saparov, W. Meng, Z. Xiao, D. B. Mitzi and Y. Yan, J. Phys. Chem. C, 2016, 120, 6435–6441 CAS.
- S. Körbel, M. A. Marques and S. Botti, J. Mater. Chem. C, 2016, 4, 3157–3167 RSC.
- J. Berry, T. Buonassisi, D. A. Egger, G. Hodes, L. Kronik, Y.-L. Loo, I. Lubomirsky, S. R. Marder, Y. Mastai and J. S. Miller, Adv. Mater., 2015, 27, 5102–5112 CrossRef CAS PubMed.
- P. P. Boix, S. Agarwala, T. M. Koh, N. Mathews and S. G. Mhaisalkar, J. Phys. Chem. Lett., 2015, 6, 898–907 CrossRef CAS PubMed.
- K. Gregor, S. Shijing and K. C. Anthony, Chem. Sci., 2014, 5, 4712–4715 RSC.
- G. Kieslich, S. Sun and T. Cheetham, Chem. Sci., 2015, 6, 3430–3433 RSC.
- W. Travis, E. Glover, H. Bronstein, D. Scanlon and R. Palgrave, Chem. Sci., 2016, 7, 4548–4556 RSC.
- T. C. Sum and N. Mathews, Energy Environ. Sci., 2014, 7, 2518–2534 CAS.
- C. C. Stoumpos and M. G. Kanatzidis, Adv. Mater., 2016, 28, 5778–5793 CrossRef CAS PubMed.
- R. E. Brandt, V. Stevanović, D. S. Ginley and T. Buonassisi, MRS Commun., 2015, 5, 265–275 CrossRef CAS.
- J. M. Frost, K. T. Butler, F. Brivio, C. H. Hendon, M. van Schilfgaarde and A. Walsh, Nano Lett., 2014, 14, 2584–2590 CrossRef CAS PubMed.
- P. Umari, E. Mosconi and F. De Angelis, Sci. Rep., 2014, 4, 4467 Search PubMed.
- L. Yu and A. Zunger, Phys. Rev. Lett., 2012, 108, 068701 CrossRef PubMed.
- A. B. Kehoe, D. O. Scanlon and G. W. Watson, Phys. Rev. B: Condens. Matter Mater. Phys., 2011, 83, 3–6 CrossRef.
- A. M. Ganose and D. O. Scanlon, J. Mater. Chem. C, 2016, 4, 1467–1475 RSC.
- S. De Wolf, J. Holovsky, S.-J. Moon, P. Löper, B. Niesen, M. Ledinsky, F.-J. Haug, J.-H. Yum and C. Ballif, J. Phys. Chem. Lett., 2014, 5, 1035–1039 CrossRef CAS PubMed.
- A. Zakutayev, C. M. Caskey, A. N. Fioretti, D. S. Ginley, J. Vidal, V. Stevanovic, E. Tea and S. Lany, J. Phys. Chem. Lett., 2014, 5, 1117–1125 CrossRef CAS PubMed.
- P. Azarhoosh, J. M. Frost, S. McKechnie, A. Walsh and M. van Schilfgaarde, APL Mater., 2016, 4, 091501 CrossRef.
- J. Mattheis, J. H. Werner and U. Rau, Phys. Rev. B: Condens. Matter Mater. Phys., 2008, 77, 085203 CrossRef.
- R. Jaramillo, M.-J. Sher, B. K. Ofori-Okai, V. Steinmann, C. Yang, K. Hartman, K. A. Nelson, A. M. Lindenberg, R. G. Gordon and T. Buonassisi, J. Appl. Phys., 2016, 119, 035101 CrossRef.
- S. D. Stranks, G. E. Eperon, G. Grancini, C. Menelaou, M. J. P. Alcocer, T. Leijtens, L. M. Herz, A. Petrozza and H. J. Snaith, Science, 2013, 342, 341–344 CrossRef CAS PubMed.
- A. E. Maughan, A. M. Ganose, M. M. Bordelon, E. M. Miller, D. O. Scanlon and J. R. Neilson, J. Am. Chem. Soc., 2016, 138, 8453–8464 CrossRef CAS PubMed.
-
R. H. Bube, Photoelectronic Properties of Semiconductors, Cambridge University Press, 1992 Search PubMed.
- Y. Kutes, L. Ye, Y. Zhou, S. Pang, B. D. Huey and N. P. Padtures, J. Phys. Chem. Lett., 2014, 5, 3335–3339 CrossRef CAS PubMed.
- S. Liu, F. Zheng, N. Z. Koocher, H. Takenaka, F. Wang and A. M. Rappe, J. Phys. Chem. Lett., 2015, 6, 693–699 CrossRef CAS PubMed.
- J. Beilsten-Edmands, G. E. Eperon, R. D. Johnson, H. J. Snaith and P. G. Radaelli, Appl. Phys. Lett., 2015, 106, 173502 CrossRef.
- K. T. Butler, J. M. Frost and A. Walsh, Energy Environ. Sci., 2015, 8, 838–848 CAS.
- M. Kepenekian, R. Robles, C. Katan, D. Sapori, L. Pedesseau and J. Even, ACS Nano, 2015, 9, 11557–11567 CrossRef CAS PubMed.
- T. Etienne, E. Mosconi and F. D. Angelis, J. Phys. Chem. Lett., 2016, 7, 1638–1645 CrossRef CAS PubMed.
- L. Yu, R. S. Kokenyesi, D. A. Keszler and A. Zunger, Adv. Energy Mater., 2013, 3, 43–48 CrossRef CAS.
- K. Leo, Nat. Nanotechnol., 2015, 10, 574–575 CrossRef CAS PubMed.
- B. Hailegnaw, S. Kirmayer, E. Edri, G. Hodes and D. Cahen, J. Phys. Chem. Lett., 2015, 6, 1543–1547 CrossRef CAS PubMed.
- K. K. Bass, R. E. McAnally, S. Zhou, P. I. Djurovich, M. E. Thompson and B. C. Melot, Chem. Commun., 2014, 50, 15819–15822 RSC.
- E. Mosconi, J. M. Azpiroz and F. De Angelis, Chem. Mater., 2015, 27, 4885–4892 CrossRef CAS.
- J. A. Christians, J. S. Manser and P. V. Kamat, J. Phys. Chem. Lett., 2015, 6, 2086–2095 CrossRef CAS PubMed.
- D. Bryant, N. Aristidou, S. Pont, I. Sanchez-Molina, T. Chotchunangatchaval, S. Wheeler, J. R. Durrant and S. A. Haque, Energy Environ. Sci., 2016, 9, 1655–1660 CAS.
- B. Conings, J. Drijkoningen, N. Gauquelin, A. Babayigit, J. D'Haen, L. D'Olieslaeger, A. Ethirajan, J. Verbeeck, J. Manca, E. Mosconi, F. D. Angelis and H.-G. Boyen, Adv. Energy Mater., 2015, 5, 1500477 CrossRef.
- E. J. Juarez-Perez, Z. Hawash, S. R. Raga, L. K. Ono and Y. Qi, Energy Environ. Sci., 2016 10.1039/C6EE02016J.
- A. Pisoni, J. Jaćimović, O. S. Barišicć, M. Spina, R. Gaál, L. Forró and E. Horváth, J. Phys. Chem. Lett., 2014, 5, 2488–2492 CrossRef CAS PubMed.
-
Y.-Y. Zhang, S. Chen, P. Xu, H. Xiang, X.-G. Gong, A. Walsh and S.-H. Wei, arXiv:1506.01301 [cond-mat.mtrl-sci], 2015.
- A. M. Ganose, C. N. Savory and D. O. Scanlon, J. Phys. Chem. Lett., 2015, 6, 4594–4598 CrossRef CAS PubMed.
- S. N. Habisreutinger, D. P. McMeekin, H. J. Snaith and R. J. Nicholas, APL Mater., 2016, 4, 091503 CrossRef.
- D. B. Mitzi, J. Chem. Soc., Dalton Trans., 2001, 1–12 RSC.
- B. Saparov and D. B. Mitzi, Chem. Rev., 2016, 116, 4558–4596 CrossRef CAS PubMed.
- A. Amat, E. Mosconi, E. Ronca, C. Quarti, P. Umari, M. K. Nazeeruddin, M. Grätzel and F. De Angelis, Nano Lett., 2014, 14, 3608–3616 CrossRef CAS PubMed.
- G. E. Eperon, S. D. Stranks, C. Menelaou, M. B. Johnston, L. M. Herz and H. J. Snaith, Energy Environ. Sci., 2014, 7, 982–988 CAS.
- C. C. Stoumpos, C. D. Malliakas and M. G. Kanatzidis, Inorg. Chem., 2013, 52, 9019–9038 CrossRef CAS PubMed.
- M. T. Weller, O. J. Weber, J. M. Frost and A. Walsh, J. Phys. Chem. Lett., 2015, 6, 3209–3212 CrossRef CAS.
- A. Binek, F. C. Hanusch, P. Docampo and T. Bein, J. Phys. Chem. Lett., 2015, 6, 1249–1253 CrossRef CAS PubMed.
- N. Pellet, P. Gao, G. Gregori, T.-Y. Yang, M. K. Nazeeruddin, J. Maier and M. Grätzel, Angew. Chem., Int. Ed., 2014, 53, 3151–3157 CrossRef CAS PubMed.
- J. Brgoch, A. Lehner, M. Chabinyc and R. Seshadri, J. Phys. Chem. C, 2014, 118, 27721–27727 CAS.
- R. J. Sutton, G. E. Eperon, L. Miranda, E. S. Parrott, B. A. Kamino, J. B. Patel, M. T. Hörantner, M. B. Johnston, A. A. Haghighirad, D. T. Moore and H. J. Snaith, Adv. Energy Mater., 2016, 6, 1502458 CrossRef.
- G. E. Eperon, G. M. Paterno, R. J. Sutton, A. Zampetti, A. A. Haghighirad, F. Cacialli and H. J. Snaith, J. Mater. Chem. A, 2015, 3, 19688–19695 CAS.
- L. Protesescu, S. Yakunin, M. I. Bodnarchuk, F. Krieg, R. Caputo, C. H. Hendon, R. X. Yang, A. Walsh and M. V. Kovalenko, Nano Lett., 2015, 15, 3692–3696 CrossRef CAS PubMed.
- K. T. Butler, J. M. Frost and A. Walsh, Mater. Horiz., 2015, 2, 228–231 RSC.
- Y. Tidhar, E. Edri, H. Weissman, D. Zohar, G. Hodes, D. Cahen, B. Rybtchinski and S. Kirmayer, J. Am. Chem. Soc., 2014, 136, 13249–13256 CrossRef CAS PubMed.
- N. Yantara, Y. Fang, S. Chen, H. A. Dewi, P. P. Boix, S. G. Mhaisalkar and N. Mathews, Chem. Mater., 2015, 27, 2309–2314 CrossRef CAS.
- J. H. Noh, S. H. Im, J. H. Heo, T. N. Mandal and S. I. Seok, Nano Lett., 2013, 13, 1764–1769 CrossRef CAS PubMed.
- N. J. Jeon, J. H. Noh, Y. C. Kim, W. S. Yang, S. Ryu and S. I. Seok, Nat. Mater., 2014, 13, 897–903 CrossRef CAS PubMed.
- W. Rehman, R. L. Milot, G. E. Eperon, C. Wehrenfennig, J. L. Boland, H. J. Snaith, M. B. Johnston and L. M. Herz, Adv. Mater., 2015, 27, 7938–7944 CrossRef CAS PubMed.
- F. Brivio, C. Caetano and A. Walsh, J. Phys. Chem. Lett., 2016, 7, 1083–1087 CrossRef CAS PubMed.
- D. P. McMeekin, G. Sadoughi, W. Rehman, G. E. Eperon, M. Saliba, M. T. Hörantner, A. Haghighirad, N. Sakai, L. Korte, B. Rech, M. B. Johnston, L. M. Herz and H. J. Snaith, Science, 2016, 351, 151–155 CrossRef CAS PubMed.
- A. Walsh, J. Mater. Chem. C, 2015, 119, 5755–5760 CAS.
- D. Umeyama, Y. Lin and H. I. Karunadasa, Chem. Mater., 2016, 28, 3241–3244 CrossRef CAS.
- T. M. Koh, K. Thirumal, H. S. Soo and N. Mathews, ChemSusChem, 2016, 9, 2541–2558 CrossRef CAS PubMed.
- D. B. Mitzi, C. D. Dimitrakopoulos and L. L. Kosbar, Chem. Mater., 2001, 13, 3728–3740 CrossRef CAS.
- D. B. Mitzi, K. Chondroudis and C. R. Kagan, IBM J. Res. Dev., 2001, 45, 29–45 CrossRef CAS.
- D. B. Mitzi, D. R. Medeiros and P. R. L. Malenfant, Inorg. Chem., 2002, 41, 2134–2145 CrossRef CAS PubMed.
- L. N. Quan, M. Yuan, R. Comin, O. Voznyy, E. M. Beauregard, S. Hoogland, A. Buin, A. R. Kirmani, K. Zhao, A. Amassian, D. H. Kim and E. H. Sargent, J. Am. Chem. Soc., 2016, 138, 2649–2655 CrossRef CAS PubMed.
- W. Jiang, J. Ying, W. Zhou, K. Shen, X. Liu, X. Gao, F. Guo, Y. Gao and T. Yang, Chem. Phys. Lett., 2016, 658, 71–75 CrossRef CAS.
- D. B. Mitzi, C. A. Feild, W. T. A. Harrison and A. M. Guloy, Nature, 1994, 369, 467–469 CrossRef CAS.
-
D. B. Mitzi, in Progress in Inorganic Chemistry, ed. K. D. Karlin, John Wiley & Sons Inc., 1999, ch. 1, vol. 48, pp. 1–121 Search PubMed.
- S. Wang, D. B. Mitzi, C. A. Feild and A. Guloys, J. Am. Chem. Soc., 1995, 117, 5297–5302 CrossRef CAS.
- D. B. Mitzi, Chem. Mater., 1996, 8, 791–800 CrossRef CAS.
- K. Chondroudis and D. B. Mitzi, Chem. Mater., 1999, 11, 3028–3030 CrossRef CAS.
- D. B. Mitzi, Inorg. Chem., 2000, 39, 6107–6113 CrossRef CAS PubMed.
- D. B. Mitzi, Chem. Mater., 2001, 13, 3283–3298 CrossRef CAS.
- N. Kitazawa and Y. Watanabe, J. Phys. Chem. Solids, 2010, 71, 797–802 CrossRef CAS.
- T. Ishihara, J. Takahashi and T. Goto, Phys. Rev. B: Condens. Matter Mater. Phys., 1990, 42, 11099–11107 CrossRef CAS.
- E. A. Muljarov, S. G. Tikhodeev, N. A. Gippius and T. Ishihara, Phys. Rev. B: Condens. Matter Mater. Phys., 1995, 51, 14370–14378 CrossRef CAS.
- N. Mercier, S. Poiroux, A. Riou and P. Batail, Inorg. Chem., 2004, 43, 8361–8366 CrossRef CAS PubMed.
- S. Sourisseau, N. Louvain, W. Bi, N. Mercier, D. Rondeau, F. Boucher, J.-Y. Buzaré and C. Legein, Chem. Mater., 2007, 19, 600–607 CrossRef CAS.
- I. C. Smith, E. T. Hoke, D. Solis-Ibarra, M. D. McGehee and H. I. Karunadasa, Angew. Chem., Int. Ed., 2014, 53, 11232–11235 CrossRef CAS PubMed.
- D. H. Cao, C. C. Stoumpos, O. K. Farha, J. T. Hupp and M. G. Kanatzidis, J. Am. Chem. Soc., 2015, 137, 7843–7850 CrossRef CAS PubMed.
- Z. Yang, C.-C. Chueh, F. Zuo, J. H. Kim, P.-W. Liang and A. K.-Y. Jen, Adv. Energy Mater., 2015, 5, 1500328 CrossRef.
- A. Dualeh, P. Gao, S. I. Seok, M. K. Nazeeruddin and M. Grätzel, Chem. Mater., 2014, 26, 6160–6164 CrossRef CAS.
- J. A. Christians, P. A. M. Herrera and P. V. Kamat, J. Am. Chem. Soc., 2015, 137, 1530–1538 CrossRef CAS PubMed.
- H. Tsai, W. Nie, J.-C. Blancon, C. C. Stoumpos, R. Asadpour, B. Harutyunyan, A. J. Neukirch, R. Verduzco, J. J. Crochet, S. Tretiak, L. Pedesseau, J. Even, M. A. Alam, G. Gupta, J. Lou, P. M. Ajayan, M. J. Bedzyk, M. G. Kanatzidis and A. D. Mohite, Nature, 2016, 536, 312–316 CrossRef CAS PubMed.
- A. Halder, R. Chulliyil, A. S. Subbiah, T. Khan, S. Chattoraj, A. Chowdhury and S. K. Sarkar, J. Phys. Chem. Lett., 2015, 6, 3483–3489 CrossRef CAS PubMed.
- W. Ke, C. Xiao, C. Wang, B. Saparov, H.-S. Duan, D. Zhao, Z. Xiao, P. Schulz, S. P. Harvey, W. Liao, W. Meng, Y. Yu, A. J. Cimaroli, C.-S. Jiang, K. Zhu, M. Al-Jassim, G. Fang, D. B. Mitzi and Y. Yan, Adv. Mater., 2016, 28, 5214–5221 CrossRef CAS PubMed.
- Y. Iwadate, K. Kawamura, K. Igarashi and J. Mochinaga, J. Phys. Chem., 1982, 86, 5205–5208 CrossRef CAS.
- Y. Chen, B. Li, W. Huang, D. Gao and Z. Liang, Chem. Commun., 2015, 51, 11997–11999 RSC.
- Q. Jiang, D. Rebollar, J. Gong, E. L. Piacentino, C. Zheng and T. Xu, Angew. Chem., Int. Ed., 2015, 54, 7617–7620 CrossRef CAS PubMed.
- M. Daub and H. Hillebrecht, Angew. Chem., Int. Ed., 2015, 54, 11016–11017 CrossRef CAS PubMed.
- Z. Xiao, W. Meng, B. Saparov, H.-S. Duan, C. Wang, C. Feng, W.-Q. Liao, W. Ke, D. Zhao, J. Wang, D. B. Mitzi and Y. Yan, J. Phys. Chem. Lett., 2016, 7, 1213–1218 CrossRef CAS PubMed.
- G. A. Mousdis, V. Gionis, G. C. Papavassiliou, C. P. Raptopoulou and A. Terzis, J. Mater. Chem., 1998, 8, 2259–2262 RSC.
- H.-B. Duan, H.-R. Zhao, X.-M. Ren, H. Zhou, Z.-F. Tian and W.-Q. Jin, Dalton Trans., 2011, 40, 1672–1683 RSC.
- S.-P. Zhao and X.-M. Ren, Dalton Trans., 2011, 40, 8261–8272 RSC.
- G.-E. Wang, X.-M. Jiang, M.-J. Zhang, H.-F. Chen, B.-W. Liu, M.-S. Wang and G.-C. Guo, CrystEngComm, 2013, 15, 10399 RSC.
- H. A. Evans, A. J. Lehner, J. G. Labram, D. H. Fabini, O. Barreda, S. R. Smock, G. Wu, M. L. Chabinyc, R. Seshadri and F. Wudl, Chem. Mater., 2016, 28, 3607–3611 CrossRef.
- A. E. Maughan, J. A. Kurzman and J. R. Neilson, Inorg. Chem., 2015, 54, 370–378 CrossRef CAS PubMed.
- D. H. Fabini, J. G. Labram, A. J. Lehner, J. S. Bechtel, H. A. Evans, A. Van der Ven, F. Wudl, M. L. Chabinyc and R. Seshadri, Inorg. Chem., 2016 DOI:acs.inorgchem.6b01539.
- B. R. Vincent, K. N. Robertson, T. S. Cameron and O. Knop, Can. J. Chem., 1987, 65, 1042–1046 CrossRef CAS.
- K. Pradeesh, M. Agarwal, K. K. Rao and G. V. Prakash, Solid State Sci., 2010, 12, 95–98 CrossRef CAS.
- E. M. Kosower, J. Org. Chem., 1964, 29, 956–957 CrossRef CAS.
- W. V. E. Doering and L. H. Knox, J. Am. Chem. Soc., 1957, 79, 352–356 CrossRef CAS.
- J.-J. Liu, Y.-F. Guan, C. Jiao, M.-J. Lin, C.-C. Huang and W. Dai, Dalton Trans., 2015, 44, 5957–5960 RSC.
- C. N. Savory, R. G. Palgrave, H. Bronstein and D. O. Scanlon, Sci. Rep., 2016, 6, 20626 CrossRef CAS PubMed.
- H. Bronstein, J. M. Frost, A. Hadipour, Y. Kim, C. B. Nielsen, R. S. Ashraf, B. P. Rand, S. Watkins and I. McCulloch, Chem. Mater., 2013, 25, 277–285 CrossRef CAS.
- W. Travis, C. E. Knapp, C. N. Savory, A. M. Ganose, P. Kafourou, X. Song, Z. Sharif, J. K. Cockcroft, D. O. Scanlon, H. Bronstein and R. G. Palgrave, Inorg. Chem., 2016, 55, 3393–3400 CrossRef CAS PubMed.
- Z. Ning, X. Gong, R. Comin, G. Walters, F. Fan, O. Voznyy, E. Yassitepe, A. Buin, S. Hoogland and E. H. Sargent, Nature, 2015, 523, 324–328 CrossRef CAS PubMed.
- J. H. Rhee, C.-C. Chung and E. W.-G. Diau, NPG Asia Mater., 2013, 5, e68 CrossRef CAS.
- J. B. Sambur, T. Novet and B. Parkinson, Science, 2010, 330, 63–66 CrossRef CAS PubMed.
- J.-W. Lee, D.-Y. Son, T. K. Ahn, H.-W. Shin, I. Y. Kim, S.-J. Hwang, M. J. Ko, S. Sul, H. Han and N.-G. Park, Sci. Rep., 2013, 3, 1050 Search PubMed.
- H. Lee, H. C. Leventis, S.-J. Moon, P. Chen, S. Ito, S. A. Haque, T. Torres, F. Nüesch, T. Geiger and S. M. Zakeeruddin, Adv. Funct. Mater., 2009, 19, 2735–2742 CrossRef CAS.
- S. H. Im, H.-j. Kim, S. W. Kim, S.-W. Kim and S. I. Seok, Energy Environ. Sci., 2011, 4, 4181–4186 CAS.
- R. Schoolar and J. Dixon, Phys. Rev., 1965, 137, A667 CrossRef.
- G. D. Scholes and G. Rumbles, Nat. Mater., 2006, 5, 683–696 CrossRef CAS PubMed.
- J. Tang, K. W. Kemp, S. Hoogland, K. S. Jeong, H. Liu, L. Levina, M. Furukawa, X. Wang, R. Debnath and D. Cha, Nat. Mater., 2011, 10, 765–771 CrossRef CAS PubMed.
- C.-H. M. Chuang, P. R. Brown, V. Bulović and M. G. Bawendi, Nat. Mater., 2014, 13, 796 CrossRef CAS PubMed.
- G. H. Carey, L. Levina, R. Comin, O. Voznyy and E. H. Sargent, Adv. Mater., 2015, 27, 3325–3330 CrossRef CAS PubMed.
- D. C. Neo, C. Cheng, S. D. Stranks, S. M. Fairclough, J. S. Kim, A. I. Kirkland, J. M. Smith, H. J. Snaith, H. E. Assender and A. A. Watt, Chem. Mater., 2014, 26, 4004–4013 CrossRef CAS.
- C. Borriello, A. Bruno, R. Diana, T. Di Luccio, P. Morvillo, R. Ricciardi, F. Villani and C. Minarini, Phys. Status Solidi A, 2015, 212, 245–251 CrossRef CAS.
- H. Lu, J. Joy, R. L. Gaspar, S. E. Bradforth and R. L. Brutchey, Chem. Mater., 2016, 28, 1897–1906 CrossRef CAS.
- L. Etgar, P. Gao, P. Qin, M. Graetzel and M. K. Nazeeruddin, J. Mater. Chem. A, 2014, 2, 11586–11590 CAS.
- L. Hu, W. Wang, H. Liu, J. Peng, H. Cao, G. Shao, Z. Xia, W. Ma and J. Tang, J. Mater. Chem. A, 2015, 3, 515–518 CAS.
- F. W. Wise, Acc. Chem. Res., 2000, 33, 773–780 CrossRef CAS PubMed.
- J. E. Murphy, M. C. Beard, A. G. Norman, S. P. Ahrenkiel, J. C. Johnson, P. Yu, O. I. Micic, R. J. Ellingson and A. J. Nozik, J. Am. Chem. Soc., 2006, 128, 3241–3247 CrossRef CAS PubMed.
- J. J. Choi, Y.-F. Lim, M. B. Santiago-Berrios, M. Oh, B.-R. Hyun, L. Sun, A. C. Bartnik, A. Goedhart, G. G. Malliaras and H. D. Abruna, Nano Lett., 2009, 9, 3749–3755 CrossRef CAS PubMed.
- J. Zhang, J. Gao, C. P. Church, E. M. Miller, J. M. Luther, V. I. Klimov and M. C. Beard, Nano Lett., 2014, 14, 6010–6015 CrossRef CAS PubMed.
- J. M. Luther, J. Gao, M. T. Lloyd, O. E. Semonin, M. C. Beard and A. J. Nozik, Adv. Mater., 2010, 22, 3704–3707 CrossRef CAS PubMed.
- L. Serrano-Lujan, N. Espinosa, T. T. Larsen-Olsen, J. Abad, A. Urbina and F. C. Krebs, Adv. Energy Mater., 2015, 5, 1501119 CrossRef.
-
Directive 2011/65/EU of the European Parliament and of the Council of 8 June 2011 on the restriction of the use of certain hazardous substances in electrical and electronic equipment (recast), http://eur-lex.europa.eu/legal-content/en/TXT/?uri=celex:32011L0065, 2011.
- Y. Han, S. Meyer, Y. Dkhissi, K. Weber, J. M. Pringle, U. Bach, L. Spiccia and Y.-B. Cheng, J. Mater. Chem. A, 2015, 3, 8139–8147 CAS.
- A. Babayigit, A. Ethirajan, M. Muller and B. Conings, Nat. Mater., 2016, 15, 247–251 CrossRef CAS PubMed.
- K. Winship, Adverse Drug React. Acute Poisoning Rev., 1987, 7, 19–38 Search PubMed.
- S. J. Lee, S. S. Shin, Y. C. Kim, D. Kim, T. K. Ahn, J. H. Noh, J. Seo and S. I. Seok, J. Am. Chem. Soc., 2016, 138, 3974–3977 CrossRef CAS PubMed.
- D. Sabba, H. K. Mulmudi, R. R. Prabhakar, T. Krishnamoorthy, T. Baikie, P. P. Boix, S. Mhaisalkar and N. Mathews, J. Phys. Chem. C, 2015, 119, 1763–1767 CAS.
- L. A. Burton and A. Walsh, J. Mater. Chem. C, 2012, 116, 24262–24267 CrossRef CAS.
- T. M. Brenner, D. A. Egger, L. Kronik, G. Hodes and D. Cahen, Nat. Rev. Mater., 2016, 1, 15007 CrossRef.
- Y. Ogomi, A. Morita, S. Tsukamoto, T. Saitho, N. Fujikawa, Q. Shen, T. Toyoda, K. Yoshino, S. S. Pandey and T. Ma, J. Phys. Chem. Lett., 2014, 5, 1004–1011 CrossRef CAS PubMed.
- F. Hao, C. C. Stoumpos, R. P. Chang and M. G. Kanatzidis, J. Am. Chem. Soc., 2014, 136, 8094–8099 CrossRef CAS PubMed.
- F. Zuo, S. T. Williams, P.-W. Liang, C.-C. Chueh, C.-Y. Liao and A. K.-Y. Jen, Adv. Mater., 2014, 26, 6454–6460 CrossRef CAS PubMed.
- Y. Li, W. Sun, W. Yan, S. Ye, H. Rao, H. Peng, Z. Zhao, Z. Bian, Z. Liu, H. Zhou and C. Huang, Adv. Energy Mater., 2016, 1601353 CrossRef.
- F. Hao, C. C. Stoumpos, D. H. Cao, R. P. H. Chang and M. G. Kanatzidis, Nat. Photonics, 2014, 8, 489–494 CrossRef CAS.
- N. K. Noel, S. D. Stranks, A. Abate, C. Wehrenfennig, S. Guarnera, A. Haghighirad, A. Sadhanala, G. E. Eperon, S. K. Pathak, M. B. Johnston, A. Petrozza, L. Herz and H. Snaith, Energy Environ. Sci., 2014, 7, 3061–3068 CAS.
- D. B. Mitzi, C. Feild, Z. Schlesinger and R. Laibowitz, J. Solid State Chem., 1995, 114, 159–163 CrossRef CAS.
- T. M. Koh, T. Krishnamoorthy, N. Yantara, C. Shi, W. L. Leong, P. P. Boix, A. C. Grimsdale, S. G. Mhaisalkar and N. Mathews, J. Mater. Chem. A, 2015, 3, 14996–15000 CAS.
- T. M. Koh, K. Fu, Y. Fang, S. Chen, T. Sum, N. Mathews, S. G. Mhaisalkar, P. P. Boix and T. Baikie, J. Mater. Chem. C, 2013, 118, 16458–16462 Search PubMed.
- I. Chung, B. Lee, J. He, R. P. Chang and M. G. Kanatzidis, Nature, 2012, 485, 486–489 CrossRef CAS PubMed.
- I. Chung, J.-H. Song, J. Im, J. Androulakis, C. D. Malliakas, H. Li, A. J. Freeman, J. T. Kenney and M. G. Kanatzidis, J. Am. Chem. Soc., 2012, 134, 8579–8587 CrossRef CAS PubMed.
- Y. Zhou, H. F. Garces, B. S. Senturk, A. L. Ortiz and N. P. Padture, Mater. Lett., 2013, 110, 127–129 CrossRef CAS.
- Z. Chen, J. J. Wang, Y. Ren, C. Yu and K. Shum, Appl. Phys. Lett., 2012, 101, 093901 CrossRef.
- M. H. Kumar, S. Dharani, W. L. Leong, P. P. Boix, R. R. Prabhakar, T. Baikie, C. Shi, H. Ding, R. Ramesh, M. Asta, M. Graetzel, S. G. Mhaisalkar and N. Mathews, Adv. Mater., 2014, 26, 7122–7127 CrossRef CAS PubMed.
- S. Dharani, H. K. Mulmudi, N. Yantara, P. T. T. Trang, N. G. Park, M. Graetzel, S. Mhaisalkar, N. Mathews and P. P. Boix, Nanoscale, 2014, 6, 1675–1679 RSC.
- M. H. Kumar, N. Yantara, S. Dharani, M. Graetzel, S. Mhaisalkar, P. P. Boix and N. Mathews, Chem. Commun., 2013, 49, 11089–11091 RSC.
- M. M. Lee, J. Teuscher, T. Miyasaka, T. N. Murakami and H. J. Snaith, Science, 2012, 338, 643–647 CrossRef CAS PubMed.
- C. Yu, Y. Ren, Z. Chen and K. Shum, J. Appl. Phys., 2013, 114, 163505 CrossRef.
- E. L. da Silva, J. M. Skelton, S. C. Parker, A. Walsh, E. L. Silva, J. M. Skelton, S. C. Parker and A. Walsh, Phys. Rev. B: Condens. Matter Mater. Phys., 2015, 91, 1–12 Search PubMed.
- R. E. Banai, H. Lee, M. A. Motyka, R. Chandrasekharan, N. J. Podraza, J. R. Brownson and M. W. Hom, IEEE J. Photovoltaics, 2013, 3, 1084–1089 CrossRef.
- D. Avellaneda, M. T. S. Nair and P. K. Nair, J. Electrochem. Soc., 2008, 155, D517–D525 CrossRef CAS.
- F. Jiang, H. Shen, W. Wang and L. Zhang, J. Electrochem. Soc., 2012, 159, H235–H238 CrossRef CAS.
- W. Albers, C. Haas, H. Vink and J. Wasscher, J. Appl. Phys., 1961, 32, 2220–2225 CrossRef CAS.
- A. Lambros, D. Geraleas and N. Economou, J. Phys. Chem. Solids, 1974, 35, 537–541 CrossRef CAS.
- H. Noguchi, A. Setiyadi, H. Tanamura, T. Nagatomo and O. Omoto, Sol. Energy Mater. Sol. Cells, 1994, 35, 325–331 CrossRef CAS.
- P. Sinsermsuksakul, L. Sun, S. W. Lee, H. H. Park, S. B. Kim, C. Yang and R. G. Gordon, Adv. Energy Mater., 2014, 4, 1400496 CrossRef.
- R. Banai, M. Horn and J. Brownson, Sol. Energy Mater. Sol. Cells, 2016, 150, 112–129 CrossRef CAS.
- G. L. Araújo and A. Mart, Sol. Energy Mater. Sol. Cells, 1994, 33, 213–240 CrossRef.
- J. Vidal, S. Lany, M. d'Avezac, A. Zunger, A. Zakutayev, J. Francis and J. Tate, Appl. Phys. Lett., 2012, 100, 032104 CrossRef.
- M. Parenteau and C. Carlone, Phys. Rev. B: Condens. Matter Mater. Phys., 1990, 41, 5227 CrossRef CAS.
- T. Sorgenfrei, F. Hofherr, T. Jauß and A. Cröll, Cryst. Res. Technol., 2013, 48, 193–199 CrossRef CAS.
- T. D. Heidel, D. Hochbaum, J. M. Sussman, V. Singh, M. E. Bahlke, I. Hiromi, J. Lee and M. A. Baldo, J. Appl. Phys., 2011, 109, 104502 CrossRef.
- K. R. Reddy, N. K. Reddy and R. Miles, Sol. Energy Mater. Sol. Cells, 2006, 90, 3041–3046 CrossRef.
- M. Sugiyama, Y. Murata, T. Shimizu, K. Ramya, C. Venkataiah, T. Sato and K. R. Reddy, Jpn. J. Appl. Phys., 2011, 50, 05FH03 CrossRef.
- G. Yue, L. Wang, X. Wang, Y. Chen and D. Peng, Nanoscale Res. Lett., 2009, 4, 359–363 CrossRef CAS PubMed.
- E. C. Greyson, J. E. Barton and T. W. Odom, Small, 2006, 2, 368–371 CrossRef CAS PubMed.
- A. Mariano and K. Chopra, Appl. Phys. Lett., 1967, 10, 282–284 CrossRef CAS.
- T. Chattopadhyay, J. Pannetier and H. Von Schnering, J. Phys. Chem. Solids, 1986, 47, 879–885 CrossRef CAS.
- D. Payne, R. Egdell, A. Walsh, G. Watson, J. Guo, P.-A. Glans, T. Learmonth and K. Smith, Phys. Rev. Lett., 2006, 96, 157403 CrossRef CAS PubMed.
- A. Walsh, D. J. Payne, R. G. Egdell and G. W. Watson, Chem. Soc. Rev., 2011, 40, 4455–4463 RSC.
- M. Ristov, G. Sinadinovski, I. Grozdanov and M. Mitreski, Thin Solid Films, 1989, 173, 53–58 CrossRef CAS.
- M. T. S. Nair and P. K. Nair, Semicond. Sci. Technol., 1991, 6, 132 CrossRef CAS.
- H.-Y. He, J. Fei and J. Lu, Mater. Sci. Semicond. Process., 2014, 24, 90–95 CrossRef CAS.
- J. Y. Kim and S. M. George, J. Mater. Chem. C, 2010, 114, 17597–17603 CAS.
- P. Sinsermsuksakul, J. Heo, W. Noh, A. S. Hock and R. G. Gordon, Adv. Energy Mater., 2011, 1, 1116–1125 CrossRef CAS.
- N. K. Reddy and K. R. Reddy, Thin Solid Films, 1998, 325, 4–6 CrossRef.
- B. Thangaraju and P. Kaliannan, J. Phys. D: Appl. Phys., 2000, 33, 1054 CrossRef CAS.
- S. Dhanapandian, A. G. Manohari, C. Manoharan, K. S. Kumar and T. Mahalingam, Mater. Sci. Semicond. Process., 2014, 18, 65–70 CrossRef CAS.
- K. Deraman, S. Sakrani, B. Ismatl, Y. Wahab and R. Gould, Int. J. Electron., 1994, 76, 917–922 CrossRef CAS.
- M. Devika, N. K. Reddy, K. Ramesh, R. Ganesan, K. Gunasekhar, E. Gopal and K. R. Reddy, J. Electrochem. Soc., 2007, 154, H67–H73 CrossRef CAS.
- A. Wangperawong, S. M. Herron, R. R. Runser, C. Hägglund, J. T. Tanskanen, B. M. Clemens and S. F. Bent, Appl. Phys. Lett., 2013, 103, 052105 CrossRef.
- A. Ghazali, Z. Zainal, M. Z. Hussein and A. Kassim, Sol. Energy Mater. Sol. Cells, 1998, 55, 237–249 CrossRef CAS.
- L. Cheng, M. Liu, M. Wang, S. Wang, G. Wang, Q. Zhou and Z. Chen, J. Alloys Compd., 2012, 545, 122–129 CrossRef CAS.
- S. Zimin, E. Gorlachev, I. Amirov, V. Naumov, G. Dubov, V. Gremenok and S. Bashkirov, Semicond. Sci. Technol., 2013, 29, 015009 CrossRef.
- T. Sajeesh, A. R. Warrier, C. S. Kartha and K. Vijayakumar, Thin Solid Films, 2010, 518, 4370–4374 CrossRef CAS.
- H. H. Park, R. Heasley, L. Sun, V. Steinmann, R. Jaramillo, K. Hartman, R. Chakraborty, P. Sinsermsuksakul, D. Chua and T. Buonassisi, Prog. Photovoltaics, 2015, 23, 901–908 CAS.
- P. Sinsermsuksakul, K. Hartman, S. B. Kim, J. Heo, L. Sun, H. H. Park, R. Chakraborty, T. Buonassisi and R. G. Gordon, Appl. Phys. Lett., 2013, 102, 053901 CrossRef.
- L. A. Burton and A. Walsh, Appl. Phys. Lett., 2013, 102, 132111 CrossRef.
- M. Patel and A. Ray, ACS Appl. Mater. Interfaces, 2014, 6, 10099–10106 CAS.
- C. Gao, H. Shen and L. Sun, Appl. Surf. Sci., 2011, 257, 6750–6755 CrossRef CAS.
- P. K. Nair, A. R. Garcia-Angelmo and M. T. S. Nair, Phys. Status Solidi A, 2015, 177, 170–177 Search PubMed.
- A. Rabkin, S. Samuha, R. E. Abutbul, V. Ezersky, L. Meshi and Y. Golan, Nano Lett., 2015, 15, 2174–2179 CrossRef CAS PubMed.
- P. K. Nair, E. Barrios-Salgado and M. T. S. Nair, Phys. Status Solidi A, 2016, 8, 2229–2236 CrossRef.
- G. Rosenfeld and E. Wallace, Arch. Ind. Hyg. Occup. Med., 1953, 8, 466–479 CAS.
- Q. Gu, Q. Pan, X. Wu, W. Shi and C. Fang, J. Cryst. Growth, 2000, 212, 605–607 CrossRef CAS.
- G. QingTian, P. QiWei, S. Wei, S. Xun and F. ChangShui, Prog. Cryst. Growth Charact. Mater., 2000, 40, 89–95 CrossRef.
- L.-C. Tang, C.-S. Chang and J. Y. Huang, J. Phys.: Condens. Matter, 2000, 12, 9129 CrossRef CAS.
- L. C. Tang, J. Y. Huang, C. Chang, M. Lee and L. Liu, J. Phys.: Condens. Matter, 2005, 17, 7275 CrossRef CAS.
- D.-K. Seo, N. Gupta, M.-H. Whangbo, H. Hillebrecht and G. Thiele, Inorg. Chem., 1998, 37, 407–410 CrossRef CAS PubMed.
- L.-C. Tang, Y.-C. Chang, J.-Y. Huang, M.-H. Lee and C.-S. Chang, Jpn. J. Appl. Phys., 2009, 48, 112402 CrossRef.
- T. Krishnamoorthy, H. Ding, C. Yan, W. L. Leong, T. Baikie, Z. Zhang, M. Sherburne, S. Li, M. Asta, N. Mathews and S. G. Mhaisalkar, J. Mater. Chem. A, 2015, 3, 23829–23832 CAS.
-
C. Huang, X. C. Yan, G. Cui, Z. Liu, S. Pang and H. Xu, Novel germanium-containing perovskite material and solar cell comprising same, CN Pat. App. CN 201410173750, 2014.
- C. C. Stoumpos, L. Frazer, D. J. Clark, Y. S. Kim, S. H. Rhim, A. J. Freeman, J. B. Ketterson, J. I. Jang and M. G. Kanatzidis, J. Am. Chem. Soc., 2015, 137, 6804–6819 CrossRef CAS PubMed.
- B. D. Malone and E. Kaxiras, Phys. Rev. B: Condens. Matter Mater. Phys., 2013, 87, 245312 CrossRef.
- Y.-Q. Zhao, L.-J. Wu, B. Liu, L.-Z. Wang, P.-B. He and M.-Q. Cai, J. Power Sources, 2016, 313, 96–103 CrossRef CAS.
- Y. Zhou, L. Wang, S. Chen, S. Qin, X. Liu, J. Chen, D.-J. Xue, M. Luo, Y. Cao, Y. Cheng, E. H. Sargent and J. Tang, Nat. Photonics, 2015, 9, 409–415 CrossRef CAS.
- L. Makinistian and E. Albanesi, J. Phys.: Condens. Matter, 2007, 19, 186211 CrossRef CAS PubMed.
- D. D. Vaughn, R. J. Patel, M. A. Hickner and R. E. Schaak, J. Am. Chem. Soc., 2010, 132, 15170–15172 CrossRef CAS PubMed.
- P. D. Antunez, J. J. Buckley and R. L. Brutchey, Nanoscale, 2011, 3, 2399 RSC.
- L. Makinistian and E. A. Albanesi, Phys. Rev. B: Condens. Matter Mater. Phys., 2006, 74, 045206 CrossRef.
- L. Makinistian and E. Albanesi, Comput. Mater. Sci., 2011, 50, 2872–2879 CrossRef CAS.
-
K. N. Abrikosov, V. Bankina, L. Poretskaya, L. Shelimova and E. Skudnova, Semiconducting II-VI, IV-VI and V-VI Compounds, Plenum Press, NY, 1969, vol. 249, p. 14 Search PubMed.
- A. Stanchev and C. Vodenicharov, Thin Solid Films, 1976, 38, 67–72 CrossRef CAS.
- S. Hao, F. Shi, V. Dravid, M. Kanatzidis and C. Wolverton, Chem. Mater., 2016, 28, 3218–3226 CrossRef CAS.
- S. Sundar and J. Chakravarty, Int. J. Environ. Res. Public Health, 2010, 7, 4267–4277 CrossRef CAS PubMed.
- B. Yang, L. Wang, J. Han, Y. Zhou, H. Song, S. Chen, J. Zhong, L. Lv, D. Niu and J. Tang, Chem. Mater., 2014, 26, 3135–3143 CrossRef CAS.
- A. Kyono, M. Kimata, M. Matsuhisa, Y. Miyashita and K. Okamoto, Phys. Chem. Miner., 2002, 29, 254–260 CrossRef CAS.
- N. W. Tideswell, F. H. Kruse and J. D. McCullough, Acta Crystallogr., 1957, 10, 99–102 CrossRef CAS.
- J. Curran, R. Philippe, J. Joseph and A. Gagnaire, Chem. Phys. Lett., 1982, 89, 511–515 CrossRef CAS.
- O. Savadogo and K. C. Mandal, J. Electrochem. Soc., 1992, 139, L16–L18 CrossRef CAS.
- O. Savadogo and K. C. Mandal, J. Electrochem. Soc., 1994, 141, 2871–2877 CrossRef CAS.
- O. Savadogo and K. C. Mandal, J. Phys. D: Appl. Phys., 1994, 27, 1070 CrossRef CAS.
- Y. Itzhaik, O. Niitsoo, M. Page and G. Hodes, J. Phys. Chem. C, 2009, 113, 4254–4256 CAS.
- J. A. Chang, J. H. Rhee, S. H. Im, Y. H. Lee, H. Jung Kim, S. I. Seok, M. K. Nazeeruddin and M. Gratzel, Nano Lett., 2010, 10, 2609–2612 CrossRef CAS PubMed.
- S.-J. Moon, Y. Itzhaik, J.-H. Yum, S. M. Zakeeruddin, G. Hodes and M. Grätzel, J. Phys. Chem. Lett., 2010, 1, 1524–1527 CrossRef CAS.
- A. M. Huerta-Flores, N. A. García-Gómez, S. M. de la Parra-Arciniega and E. M. Sánchez, Semicond. Sci. Technol., 2016, 31, 085011 CrossRef.
- K. Tsujimoto, D.-C. Nguyen, S. Ito, H. Nishino, H. Matsuyoshi, A. Konno, G. R. A. Kumara and K. Tennakone, J. Phys. Chem. C, 2012, 116, 13465–13471 CAS.
- J. A. Chang, S. H. Im, Y. H. Lee, H.-J. Kim, C.-S. Lim, J. H. Heo and S. I. Seok, Nano Lett., 2012, 12, 1863–1867 CrossRef CAS PubMed.
- Y. C. Choi, D. U. Lee, J. H. Noh, E. K. Kim and S. I. Seok, Adv. Funct. Mater., 2014, 24, 3587–3592 CrossRef CAS.
- Y. C. Choi and S. I. Seok, Adv. Funct. Mater., 2015, 25, 2892–2898 CrossRef CAS.
- V. Janošević, M. Mitrić, N. Bundaleski, Z. Rakočević and I. L. Validžić, Prog. Photovoltaics, 2015, 24, 704–715 Search PubMed.
- M. Y. Versavel and J. A. Haber, Thin Solid Films, 2007, 515, 7171–7176 CrossRef CAS.
- R. Mane and C. Lokhande, Mater. Chem. Phys., 2003, 82, 347–354 CrossRef CAS.
- I. Grozdanov, Semicond. Sci. Technol., 1994, 9, 1234 CrossRef CAS.
- K. C. Godel, Y. C. Choi, B. Roose, A. Sadhanala, H. J. Snaith, S. I. Seok, U. Steiner and S. K. Pathak, Chem. Commun., 2015, 51, 8640–8643 RSC.
- R. X. Yang, K. T. Butler and A. Walsh, J. Phys. Chem. Lett., 2015, 6, 5009–5014 CrossRef CAS PubMed.
- T. B. Nasr, H. Maghraoui-Meherzi, H. B. Abdallah and R. Bennaceur, Phys. B, 2011, 406, 287–292 CrossRef.
- R. Caracas and X. Gonze, Phys. Chem. Miner., 2005, 32, 295–300 CrossRef CAS.
- M. R. Filip, C. E. Patrick and F. Giustino, Phys. Rev. B: Condens. Matter Mater. Phys., 2013, 87, 205125 CrossRef.
- J. Ma, Y. Wang, Y. Wang, P. Peng, J. Lian, X. Duan, Z. Liu, X. Liu, Q. Chen, T. Kim, G. Yao and W. Zheng, CrystEngComm, 2011, 13, 2369–2374 RSC.
- K. Rajpure and C. Bhosale, Mater. Chem. Phys., 2002, 73, 6–12 CrossRef CAS.
- Y. Lai, Z. Chen, C. Han, L. Jiang, F. Liu, J. Li and Y. Liu, Appl. Surf. Sci., 2012, 261, 510–514 CrossRef CAS.
- C. Chen, W. Li, Y. Zhou, C. Chen, M. Luo, X. Liu, K. Zeng, B. Yang, C. Zhang, J. Han and J. Tang, Appl. Phys. Lett., 2015, 107, 043905 CrossRef.
- J. Yang, Y. Lai, Y. Fan, Y. Jiang, D. Tang, L. Jiang, F. Liu and J. Li, RSC Adv., 2015, 5, 85592–85597 RSC.
- J. J. Carey, J. P. Allen, D. O. Scanlon and G. W. Watson, J. Solid State Chem., 2014, 213, 116–125 CrossRef CAS.
- C. Bhosale, M. Uplane, P. Patil and C. Lockhande, Thin Solid Films, 1994, 248, 137–139 CrossRef CAS.
- C. E. Patrick and F. Giustino, Adv. Funct. Mater., 2011, 21, 4663–4667 CrossRef CAS.
- Y. C. Choi, T. N. Mandal, W. S. Yang, Y. H. Lee, S. H. Im, J. H. Noh and S. I. Seok, Angew. Chem., Int. Ed., 2014, 53, 1329–1333 CrossRef CAS PubMed.
- M. Luo, M. Leng, X. Liu, J. Chen, C. Chen, S. Qin and J. Tang, Appl. Phys. Lett., 2014, 104, 173904 CrossRef.
- Y. Zhou, M. Leng, Z. Xia, J. Zhong, H. Song, X. Liu, B. Yang, J. Zhang, J. Chen, K. Zhou, J. Han, Y. Cheng and J. Tang, Adv. Energy Mater., 2014, 4, 1301846 CrossRef.
- M. Leng, M. Luo, C. Chen, S. Qin, J. Chen, J. Zhong and J. Tang, Appl. Phys. Lett., 2014, 105, 083905 CrossRef.
- X. Liu, C. Chen, L. Wang, J. Zhong, M. Luo, J. Chen, D.-J. Xue, D. Li, Y. Zhou and J. Tang, Prog. Photovoltaics, 2015, 23, 1828–1836 CAS.
- S. Messina, M. T. S. Nair and P. K. Nair, Thin Solid Films, 2007, 515, 5777–5782 CrossRef CAS.
- S. Messina, M. T. S. Nair and P. K. Nair, J. Electrochem. Soc., 2009, 156, H327–H332 CrossRef CAS.
- Y. C. Choi, Y. H. Lee, S. H. Im, J. H. Noh, T. N. Mandal, W. S. Yang and S. I. Seok, Adv. Energy Mater., 2014, 4, 1301680 CrossRef.
- A. Kikuchi, Y. Oka and E. Sawaguchi, J. Phys. Soc. Jpn., 1967, 23, 337–354 CrossRef CAS.
- E. Fatuzzo, G. Harbeke, W. J. Merz, R. Nitsche, H. Roetschi and W. Ruppel, Phys. Rev., 1962, 127, 2036–2037 CrossRef CAS.
- P. Kwolek, K. Pilarczyk, T. Tokarski, J. Mech, J. Irzmański and K. Szaciłlowski, Nanotechnology, 2015, 26, 105710 CrossRef PubMed.
- K. T. Butler, S. McKechnie, P. Azarhoosh, M. van Schilfgaarde, D. O. Scanlon and A. Walsh, Appl. Phys. Lett., 2016, 108, 112103 CrossRef.
- Y. Rodrìguez-Lazcano, M. T. S. Nair and P. K. Nair, J. Electrochem. Soc., 2005, 152, G635–G638 CrossRef.
- C. Garza, S. Shaji, A. Arato, E. P. Tijerina, G. A. Castillo, T. D. Roy and B. Krishnan, Sol. Energy Mater. Sol. Cells, 2011, 95, 2001–2005 CrossRef CAS.
- W. Wubet and D.-H. Kuo, Mater. Res. Bull., 2014, 53, 290–294 CrossRef CAS.
- S. Manolache, A. Duta, L. Isac, M. Nanu, A. Goossens and J. Schoonman, Thin Solid Films, 2007, 515, 5957–5960 CrossRef CAS.
- J. Zhou, G.-Q. Bian, Q.-Y. Zhu, Y. Zhang, C.-Y. Li and J. Dai, J. Solid State Chem., 2009, 182, 259–264 CrossRef CAS.
- D. Colombara, L. Peter, K. Rogers, J. Painter and S. Roncallo, Thin Solid Films, 2011, 519, 7438–7443 CrossRef CAS.
- A. Kyono and M. Kimata, Am. Mineral., 2005, 90, 162–165 CrossRef CAS.
- M. Kumar and C. Persson, Energy Procedia, 2014, 44, 176–183 CrossRef CAS.
- T. Maeda and T. Wada, Thin Solid Films, 2015, 582, 401–407 CrossRef CAS.
- W. Septina, S. Ikeda, Y. Iga, T. Harada and M. Matsumura, Thin Solid Films, 2014, 550, 700–704 CrossRef CAS.
- Y. C. Choi, E. J. Yeom, T. K. Ahn and S. I. Seok, Angew. Chem., Int. Ed., 2015, 54, 4005–4009 CrossRef CAS PubMed.
- S. Banu, S. J. Ahn, S. K. Ahn, K. Yoon and A. Cho, Sol. Energy Mater. Sol. Cells, 2016, 151, 14–23 CrossRef CAS.
- D.-J. Xue, B. Yang, Z.-K. Yuan, G. Wang, X. Liu, Y. Zhou, L. Hu, D. Pan, S. Chen and J. Tang, Adv. Energy Mater., 2015, 5, 1501203 CrossRef.
- A. W. Welch, L. L. Baranowski, P. Zawadzki, S. Lany, C. A. Wolden and A. Zakutayev, Appl. Phys. Express, 2015, 8, 082301 CrossRef.
- F. W. de Souza Lucas, A. W. Welch, L. L. Baranowski, P. C. Dippo, H. Hempel, T. Unold, R. Eichberger, B. Blank, U. Rau, L. H. Mascaro and A. Zakutayev, J. Phys. Chem. C, 2016, 120, 18377–18385 CAS.
- L. Wan, C. Ma, K. Hu, R. Zhou, X. Mao, S. Pan, L. H. Wong and J. Xu, J. Alloys Compd., 2016, 680, 182–190 CrossRef CAS.
- B. Saparov, F. Hong, J.-P. Sun, H.-S. Duan, W. Meng, S. Cameron, I. G. Hill, Y. Yan and D. B. Mitzi, Chem. Mater., 2015, 27, 5622–5632 CrossRef CAS.
- G. Bator, R. Jakubas, J. Baran and H. Ratajczak, Vib. Spectrosc., 1995, 8, 425–433 CrossRef CAS.
- C. Pawlaczyk, R. Jakubas and H.-G. Unruh, Solid State Commun., 1998, 108, 247–250 CrossRef CAS.
- J.-C. Hebig, I. Kühn, J. Flohre and T. Kirchartz, ACS Energy Lett., 2016, 1, 309–314 CrossRef CAS.
- P. Szklarz, A. Pietraszko, R. Jakubas, G. Bator, P. Zieliński and M. Gałazka, J. Phys.: Condens. Matter, 2008, 20, 255221 CrossRef.
- C.-Y. Mao, W.-Q. Liao, Z.-X. Wang, P.-F. Li, X.-H. Lv, H.-Y. Ye and Y. Zhang, Dalton Trans., 2016, 45, 5229–5233 RSC.
- W. Serfontein and R. Mekel, Res. Commun. Chem. Pathol. Pharmacol., 1979, 26, 391–411 CAS.
- A. Slikkerveer and F. A. de Wolff, Med. Toxicol. Adverse Drug Exper., 1989, 4, 303–323 CrossRef CAS.
- N. M. Leonard, L. C. Wieland and R. S. Mohan, Tetrahedron, 2002, 58, 8373–8397 CrossRef CAS.
- R. Mohan, Nat. Chem., 2010, 2, 336 CrossRef CAS PubMed.
- P. Pramanik and R. N. Bhattacharya, J. Electrochem. Soc., 1980, 127, 2087 CrossRef CAS.
- R. N. Bhattacharya and P. Pramanik, J. Electrochem. Soc., 1982, 129, 332–335 CrossRef CAS.
- Y. Xia, D. Qian, D. Hsieh, L. Wray, A. Pal, H. Lin, A. Bansil, D. Grauer, Y. S. Hor, R. J. Cava and M. Z. Hasan, Nat. Phys., 2009, 5, 398–402 CrossRef CAS.
- M. A. Tumelero, L. C. Benetti, E. Isoppo, R. Faccio, G. Zangari and A. A. Pasa, J. Phys. Chem. C, 2016, 120, 11797–11806 CAS.
- R. Mane and C. Lokhande, Mater. Chem. Phys., 2000, 65, 1–31 CrossRef CAS.
- P. Sonawane and L. Patil, Mater. Chem. Phys., 2007, 105, 157–161 CrossRef CAS.
- W. Liu, H. Ji, J. Wang, X. Zheng, J. Lai, J. Ji, T. Li, Y. Ma, H. Li, S. Zhao and Z. Jin, J. Mater. Sci.: Mater. Electron., 2014, 26, 1474–1484 CrossRef.
- M. E. Rincón, M. Sánchez, P. J. George, A. Sánchez and P. K. Nair, J. Solid State Chem., 1998, 136, 167–174 CrossRef.
- H. Song, X. Zhan, D. Li, Y. Zhou, B. Yang, K. Zeng, J. Zhong, X. Miao and J. Tang, Sol. Energy Mater. Sol. Cells, 2016, 146, 1–7 CrossRef CAS.
- H. Moreno-García, M. T. S. Nair and P. K. Nair, Thin Solid Films, 2011, 519, 2287–2295 CrossRef.
- M. Calixto-Rodriguez, H. M. García, M. T. S. Nair and P. K. Nair, ECS J. Solid State Sci. Technol., 2013, 2, Q69–Q73 CrossRef CAS.
- S. ten Haaf, H. Sträter, R. Brüggemann, G. H. Bauer, C. Felser and G. Jakob, Thin Solid Films, 2013, 535, 394–397 CrossRef CAS.
- M. A. Tumelero, R. Faccio and A. A. Pasa, J. Phys. Chem. C, 2016, 120, 1390–1399 CAS.
- D. Becerra, M. T. S. Nair and P. K. Nair, J. Electrochem. Soc., 2011, 158, H741–H749 CrossRef CAS.
- A. K. Rath, M. Bernechea, L. Martinez and G. Konstantatos, Adv. Mater., 2011, 23, 3712–3717 CrossRef CAS PubMed.
- A. K. Rath, M. Bernechea, L. Martinez, F. P. G. de Arquer, J. Osmond and G. Konstantatos, Nat. Photonics, 2012, 6, 529–534 CrossRef CAS.
- L. M. Peter, K. G. U. Wijayantha, D. J. Riley and J. P. Waggett, J. Phys. Chem. B, 2003, 107, 8378–8381 CrossRef CAS.
- D. Esparza, I. Zarazúa, T. López-Luke, R. Carriles, A. Torres-Castro and E. D. la Rosa, Electrochim. Acta, 2015, 180, 486–492 CrossRef CAS.
- H. Bao, X. Cui, C. M. L. Y. Gan, J. Zhang and J. Guo, J. Phys. Chem. C, 2007, 111, 12279–12283 CAS.
- H.-C. Liao, M.-C. Wu, M.-H. Jao, C.-M. Chuang, Y.-F. Chen and W.-F. Su, CrystEngComm, 2012, 14, 3645–3652 RSC.
- Y. Cao, M. Bernechea, A. Maclachlan, V. Zardetto, M. Creatore, S. A. Haque and G. Konstantatos, Chem. Mater., 2015, 27, 3700–3706 CrossRef CAS.
- L. Martinez, M. Bernechea, F. P. G. de Arquer and G. Konstantatos, Adv. Energy Mater., 2011, 1, 1029–1035 CrossRef CAS.
- L. Martinez, A. Stavrinadis, S. Higuchi, S. L. Diedenhofen, M. Bernechea, K. Tajima and G. Konstantatos, Phys. Chem. Chem. Phys., 2013, 15, 5482–5487 RSC.
- L. Whittaker-Brooks, J. Gao, A. K. Hailey, C. R. Thomas, N. Yao and Y.-L. Loo, J. Mater. Chem. C, 2015, 3, 2686–2692 RSC.
- M. Aresti, M. Saba, R. Piras, D. Marongiu, G. Mula, F. Quochi, A. Mura, C. Cannas, M. Mureddu, A. Ardu, G. Ennas, V. Calzia, A. Mattoni, A. Musinu and G. Bongiovanni, Adv. Funct. Mater., 2014, 24, 3341–3350 CrossRef CAS.
- M. Bernechea, Y. Cao and G. Konstantatos, J. Mater. Chem. A, 2015, 3, 20642–20648 CAS.
- A. J. Lehner, D. H. Fabini, H. A. Evans, C.-A. Hébert, S. R. Smock, J. Hu, H. Wang, J. W. Zwanziger, M. L. Chabinyc and R. Seshadri, Chem. Mater., 2015, 27, 7137–7148 CrossRef CAS.
- A. J. Lehner, H. Wang, D. H. Fabini, C. D. Liman, C.-A. Hébert, E. E. Perry, M. Wang, G. C. Bazan, M. L. Chabinyc and R. Seshadri, Appl. Phys. Lett., 2015, 107, 131109 CrossRef.
- A. M. Ganose, K. T. Butler, A. Walsh and D. O. Scanlon, J. Mater. Chem. A, 2016, 4, 2060–2068 CAS.
- H. Han, M. Hong, S. S. Gokhale, S. B. Sinnott, K. Jordan, J. E. Baciak and J. C. Nino, J. Phys. Chem. C, 2014, 118, 3244–3250 CAS.
- D. Nason and L. Keller, J. Cryst. Growth, 1995, 156, 221–226 CrossRef CAS.
- K. M. Boopathi, S. Raman, R. Mohanraman, F.-C. Chou, Y.-Y. Chen, C.-H. Lee, F.-C. Chang and C.-W. Chu, Sol. Energy Mater. Sol. Cells, 2014, 121, 35–41 CrossRef CAS.
- M. Ruck, Z. Kristallogr., 1995, 210, 650–655 CAS.
- D. J. Singh, Phys. Rev. B: Condens. Matter Mater. Phys., 2010, 82, 155145 CrossRef.
- G. E. Jellison, J. O. Ramey and L. A. Boatner, Phys. Rev. B: Condens. Matter Mater. Phys., 1999, 59, 9718–9721 CrossRef CAS.
- N. J. Podraza, W. Qiu, B. B. Hinojosa, H. Xu, M. A. Motyka, S. R. Phillpot, J. E. Baciak, S. Trolier-McKinstry and J. C. Nino, J. Appl. Phys., 2013, 114, 033110 CrossRef.
- H. Yorikawa and S. Muramatsu, J. Phys.: Condens. Matter, 2008, 20, 325220 CrossRef.
- R. E. Brandt, R. C. Kurchin, R. L. Z. Hoye, J. R. Poindexter, M. W. B. Wilson, S. Sulekar, F. Lenahan, P. X. T. Yen, V. Stevanović, J. C. Nino, M. G. Bawendi and T. Buonassisi, J. Phys. Chem. Lett., 2015, 6, 4297–4302 CrossRef CAS PubMed.
- F. Ma, M. Zhou, Y. Jiao, G. Gao, Y. Gu, A. Bilic, Z. Chen and A. Du, Sci. Rep., 2015, 5, 17558 CrossRef CAS PubMed.
- E. Dönges, Z. Anorg. Allg. Chem., 1950, 263, 112–132 CrossRef.
- W. Haase-Wessel, Naturwissenschaften, 1973, 60, 474 CrossRef CAS.
- T. P. Braun and F. J. DiSalvo, Acta Crystallogr., Sect. C: Cryst. Struct. Commun., 2000, 56, e1–e2 Search PubMed.
- D.-W. Shin, S.-C. Hyun, S. an Park, Y.-G. Kim, C. dae Kim and W.-T. Kim, J. Phys. Chem. Solids, 1994, 55, 825–830 CrossRef CAS.
- R. Nitsche and W. Merz, J. Phys. Chem. Solids, 1960, 13, 154–155 CrossRef CAS.
- J. Horák and K. Čermák, Cechoslovackij fiziceskij zurnal B, 1965, 15, 536–538 Search PubMed.
- N. T. Hahn, J. L. Self and C. B. Mullins, J. Phys. Chem. Lett., 2012, 3, 1571–1576 CrossRef CAS PubMed.
- N. T. Hahn, A. J. E. Rettie, S. K. Beal, R. R. Fullon and C. B. Mullins, J. Phys. Chem. C, 2012, 116, 24878–24886 CAS.
- H. Shi, W. Ming and M.-H. Du, Phys. Rev. B: Condens. Matter Mater. Phys., 2016, 93, 104108 CrossRef.
- K.-L. Zhang, C.-M. Liu, F.-Q. Huang, C. Zheng and W.-D. Wang, Appl. Catal., B, 2006, 68, 125–129 CrossRef CAS.
- J. Zhang, F. Shi, J. Lin, D. Chen, J. Gao, Z. Huang, X. Ding and C. Tang, Chem. Mater., 2008, 20, 2937–2941 CrossRef CAS.
- H. Cheng, B. Huang and Y. Dai, Nanoscale, 2014, 6, 2009–2026 RSC.
- D. S. Bhachu, S. J. A. Moniz, S. Sathasivam, D. O. Scanlon, A. Walsh, S. M. Bawaked, M. Mokhtar, A. Y. Obaid, I. P. Parkin, J. Tang and C. J. Carmalt, Chem. Sci., 2016, 7, 4832–4841 RSC.
- A. M. Ganose, M. Cuff, K. T. Butler, A. Walsh and D. O. Scanlon, Chem. Mater., 2016, 28, 1980–1984 CrossRef CAS PubMed.
- L. Zhao, X. Zhang, C. Fan, Z. Liang and P. Han, Phys. B, 2012, 407, 3364–3370 CrossRef CAS.
- S. Poznyak and A. Kulak, Electrochim. Acta, 1990, 35, 1941–1947 CrossRef CAS.
- K. Zhao, X. Zhang and L. Zhang, Electrochem. Commun., 2009, 11, 612–615 CrossRef CAS.
- K. Wang, F. Jia, Z. Zheng and L. Zhang, Electrochem. Commun., 2010, 12, 1764–1767 CrossRef CAS.
- S. Sfaelou, D. Raptis, V. Dracopoulos and P. Lianos, RSC Adv., 2015, 5, 95813–95816 RSC.
- M. Fang, H. Jia, W. He, Y. Lei, L. Zhang and Z. Zheng, Phys. Chem. Chem. Phys., 2015, 17, 13531–13538 RSC.
- A. Luz, J. Conradt, M. Wolff, H. Kalt and C. Feldmann, Solid State Sci., 2013, 19, 172–177 CrossRef CAS.
- D. J. Temple, A. B. Kehoe, J. P. Allen, G. W. Watson and D. O. Scanlon, J. Phys. Chem. C, 2012, 116, 7334–7340 CAS.
- M. Kumar and C. Persson, J. Renewable Sustainable Energy, 2013, 5, 031616 CrossRef.
- D. Parker and D. J. Singh, Phys. Rev. B: Condens. Matter Mater. Phys., 2011, 83, 233206 CrossRef.
- C. Tablero, J. Phys. Chem. C, 2015, 119, 8857–8863 CAS.
- S. H. Pawar, A. J. Pawar and P. N. Bhosale, Bull. Mater. Sci., 1986, 8, 423–426 CrossRef CAS.
- P. Sonawane, P. Wani, L. Patil and T. Seth, Mater. Chem. Phys., 2004, 84, 221–227 CrossRef CAS.
- V. Balasubramanian, N. Suriyanarayanan, S. Prabahar, S. Srikanth and P. Ravi, Optoelectron. Adv. Mater., Rapid Commun., 2012, 6, 104–106 CAS.
- W. Wubet, D.-H. Kuo and H. Abdullah, J. Solid State Chem., 2015, 230, 237–242 CrossRef CAS.
- V. Estrella, M. T. S. Nair and P. K. Nair, Semicond. Sci. Technol., 2003, 18, 190 CrossRef CAS.
- N. J. Gerein and J. A. Haber, Chem. Mater., 2006, 18, 6297–6302 CrossRef CAS.
- F. Mesa, A. Dussan and G. Gordillo, Phys. B, 2009, 404, 5227–5230 CrossRef CAS.
- F. Mesa, G. Gordillo, T. Dittrich, K. Ellmer, R. Baier and S. Sadewasser, Appl. Phys. Lett., 2010, 96, 082113 CrossRef.
- M. Yakushev, P. Maiello, T. Raadik, M. Shaw, P. Edwards, J. Krustok, A. Mudryi, I. Forbes and R. Martin, Thin Solid Films, 2014, 562, 195–199 CrossRef CAS.
- A. B. Kehoe, D. J. Temple, G. W. Watson and D. O. Scanlon, Phys. Chem. Chem. Phys., 2013, 15, 15477–15484 RSC.
- M. Kumar and C. Persson, Appl. Phys. Lett., 2013, 102, 062109 CrossRef.
- C. Tablero, Prog. Photovoltaics, 2013, 21, 894–899 CAS.
- J. Yin and J. Jia, CrystEngComm, 2014, 16, 2795–2801 RSC.
- X. Liu, H. Zheng, J. Zhang, Y. Xiao and Z. Wang, J. Mater. Chem. A, 2013, 1, 10703–10712 CAS.
- S. Geller and J. H. Wernick, Acta Crystallogr., 1959, 12, 46–54 CrossRef CAS.
- P.-C. Huang, W.-C. Yang and M.-W. Lee, J. Phys. Chem. C, 2013, 117, 18308–18314 CAS.
- K. Hoang, S. D. Mahanti, J. R. Salvador and M. G. Kanatzidis, Phys. Rev. Lett., 2007, 99, 156403 CrossRef PubMed.
- S. N. Guin, S. Banerjee, D. Sanyal, S. K. Pati and K. Biswas, Inorg. Chem., 2016, 55, 6323–6331 CrossRef CAS PubMed.
- B. Pejova, I. Grozdanov, D. Nesheva and A. Petrova, Chem. Mater., 2008, 20, 2551–2565 CrossRef CAS.
- B. Pejova, D. Nesheva, Z. Aneva and A. Petrova, J. Phys. Chem. C, 2011, 115, 37–46 CAS.
- C. Chen, X. Qiu, S. Ji, C. Jia and C. Ye, CrystEngComm, 2013, 15, 7644–7648 RSC.
- S. Zhou, J. Yang, W. Li, Q. Jiang, Y. Luo, D. Zhang, Z. Zhou and X. Li, J. Electrochem. Soc., 2016, 163, D63–D67 CrossRef CAS.
- N. Liang, W. Chen, F. Dai, X. Wu, W. Zhang, Z. Li, J. Shen, S. Huang, Q. He, J. Zai, N. Fang and X. Qian, CrystEngComm, 2015, 17, 1902–1905 RSC.
- M. Bernechea, N. C. Miller, G. Xercavins, D. So, A. Stavrinadis and G. Konstantatos, Nat. Photonics, 2016, 10, 521–525 CrossRef CAS.
- P. Fourcroy, M. Palazzi, J. Rivet, J. Flahaut and R. Céolin, Mater. Res. Bull., 1979, 14, 325–328 CrossRef CAS.
- Y. Kim, Z. Yang, A. Jain, O. Voznyy, G.-H. Kim, M. Liu, L. N. Quan, F. P. García de Arquer, R. Comin, J. Z. Fan and E. H. Sargent, Angew. Chem., Int. Ed., 2016, 55, 9586 CrossRef CAS PubMed.
- S. V. Mel'nikova and A. I. Zaitsev, Phys. Solid State, 1997, 39, 1652–1654 CrossRef.
- A. Jorio, R. Currat, D. Myles, G. McIntyre, I. Aleksandrova, J. Kiat and P. Saint-Grégoire, Phys. Rev. B: Condens. Matter Mater. Phys., 2000, 61, 3857–3862 CrossRef CAS.
- F. V. Motsnyi, O. M. Smolanka, V. I. Sugakov and E. Y. Peresh, Solid State Commun., 2006, 137, 221–224 CrossRef CAS.
- G. Bator, J. Baran, R. Jakubas and L. Sobczyk, J. Mol. Struct., 1998, 450, 89–100 CrossRef CAS.
- K. K. Bass, L. Estergreen, C. N. Savory, J. Buckeridge, D. O. Scanlon, P. I. Djurovich, S. E. Bradforth, M. E. Thompson and B. C. Melot, Inorg. Chem., 2016 DOI:10.1021/acs.inorgchem.6b01571.
- O. Lindquist, Acta Chem. Scand., 1968, 22, 2943–2952 CrossRef.
- B. Chabot and E. Parthé, Acta Crystallogr., Sect. B: Struct. Sci., 1978, 34, 645–648 CrossRef.
- K. Eckhardt, V. Bon, J. Getzschmann, J. Grothe, F. M. Wisser and S. Kaskel, Chem. Commun., 2016, 52, 3058–3060 RSC.
- B.-W. Park, B. Philippe, X. Zhang, H. Rensmo, G. Boschloo and E. M. J. Johansson, Adv. Mater., 2015, 27, 6806–6813 CrossRef CAS PubMed.
- M. Lyu, J.-H. Yun, M. Cai, Y. Jiao, P. V. Bernhardt, M. Zhang, Q. Wang, A. Du, H. Wang, G. Liu and L. Wang, Nano Res., 2016, 9, 692–702 CrossRef CAS.
- T. Singh, A. Kulkarni, M. Ikegami and T. Miyasaka, ACS Appl. Mater. Interfaces, 2016, 8, 14542–14547 CAS.
- S. Öz, J.-C. Hebig, E. Jung, T. Singh, A. Lepcha, S. Olthof, F. Jan, Y. Gao, R. German, P. H. van Loosdrecht, K. Meerholz, T. Kirchartz and S. Mathur, Sol. Energy Mater. Sol. Cells, 2016 DOI:10.1016/j.solmat.2016.01.035.
- R. L. Z. Hoye, R. E. Brandt, A. Osherov, V. Stevanović, S. D. Stranks, M. W. B. Wilson, H. Kim, A. J. Akey, J. D. Perkins, R. C. Kurchin, J. R. Poindexter, E. N. Wang, M. G. Bawendi, V. Bulović and T. Buonassisi, Chem. – Eur. J., 2016, 22, 2605–2610 CrossRef CAS PubMed.
- T. Kawai and S. Shimanuki, Phys. Status Solidi B, 1993, 177, K43–K45 CrossRef CAS.
- G. Volonakis, M. R. Filip, A. A. Haghighirad, N. Sakai, B. Wenger, H. J. Snaith and F. Giustino, J. Phys. Chem. Lett., 2016, 7, 1254–1259 CrossRef CAS PubMed.
- A. H. Slavney, T. Hu, A. M. Lindenberg and H. I. Karunadasa, J. Am. Chem. Soc., 2016, 138, 2138–2141 CrossRef CAS PubMed.
- D. M. Fabian and S. Ardo, J. Mater. Chem. A, 2016, 4, 6837–6841 CAS.
- C. Roldan-Carmona, P. Gratia, I. Zimmermann, G. Grancini, P. Gao, M. Graetzel and M. K. Nazeeruddin, Energy Environ. Sci., 2015, 8, 3550–3556 CAS.
- M. Saliba, T. Matsui, J.-Y. Seo, K. Domanski, J.-P. Correa-Baena, M. K. Nazeeruddin, S. M. Zakeeruddin, W. Tress, A. Abate, A. Hagfeldt and M. Grätzel, Energy Environ. Sci., 2016, 9, 1989–1997 CAS.
- W. S. Yang, J. H. Noh, N. J. Jeon, Y. C. Kim, S. Ryu, J. Seo and S. I. Seok, Science, 2015, 348, 1234–1237 CrossRef CAS PubMed.
- Y.-H. Huang, R. I. Dass, Z.-L. Xing and J. B. Goodenough, Science, 2006, 312, 254–257 CrossRef CAS PubMed.
- S. Sengodan, S. Choi, A. Jun, T. H. Shin, Y.-W. Ju, H. Y. Jeong, J. Shin, J. T. S. Irvine and G. Kim, Nat. Mater., 2015, 14, 205–209 CrossRef CAS PubMed.
- L. R. Morrs and W. R. Robinson, Acta Crystallogr., Sect. B: Struct. Sci., 1972, 28, 653–654 CrossRef.
- F. Pelle, B. Jacquier, J. Denis and B. Blanzat, J. Lumin., 1978, 17, 61–72 CrossRef CAS.
- G. Giorgi and K. Yamashita, Chem. Lett., 2015, 44, 826–828 CrossRef CAS.
- A. Kojima, K. Teshima, Y. Shirai and T. Miyasaka, J. Am. Chem. Soc., 2009, 131, 6050–6051 CrossRef CAS PubMed.
- J.-H. Im, C.-R. Lee, J.-W. Lee, S.-W. Park and N.-G. Park, Nanoscale, 2011, 3, 4088–4093 RSC.
- J. Burschka, N. Pellet, S.-J. Moon, R. Humphry-Baker, P. Gao, M. K. Nazeeruddin and M. Grätzel, Nature, 2013, 499, 316–319 CrossRef CAS PubMed.
- J. H. Heo, H. J. Han, D. Kim, T. K. Ahn and S. H. Im, Energy Environ. Sci., 2015, 8, 1602–1608 CAS.
- Q. Dong, Y. Yuan, Y. Shao, Y. Fang, Q. Wang and J. Huang, Energy Environ. Sci., 2015, 8, 2464–2470 CAS.
- M. Kulbak, D. Cahen and G. Hodes, J. Phys. Chem. Lett., 2015, 6, 2452–2456 CrossRef CAS PubMed.
-
Research Cell Efficiency Records (NREL), http://www.nrel.gov/ncpv/, accessed: July 22, 2016.
- E. J. Klem, D. D. MacNeil, P. W. Cyr, L. Levina and E. H. Sargent, Appl. Phys. Lett., 2007, 90, 183113 CrossRef.
- J. M. Luther, M. Law, M. C. Beard, Q. Song, M. O. Reese, R. J. Ellingson and A. J. Nozik, Nano Lett., 2008, 8, 3488–3492 CrossRef CAS PubMed.
- W. Ma, J. M. Luther, H. Zheng, Y. Wu and A. P. Alivisatos, Nano Lett., 2009, 9, 1699–1703 CrossRef CAS PubMed.
- R. Debnath, J. Tang, D. A. Barkhouse, X. Wang, A. G. Pattantyus-Abraham, L. Brzozowski, L. Levina and E. H. Sargent, J. Am. Chem. Soc., 2010, 132, 5952–5953 CrossRef CAS PubMed.
- W. Ma, S. L. Swisher, T. Ewers, J. Engel, V. E. Ferry, H. A. Atwater and A. P. Alivisatos, ACS Nano, 2011, 5, 8140–8147 CrossRef CAS PubMed.
- A. H. Ip, S. M. Thon, S. Hoogland, O. Voznyy, D. Zhitomirsky, R. Debnath, L. Levina, L. R. Rollny, G. H. Carey and A. Fischer,
et al.
, Nat. Nanotechnol., 2012, 7, 577–582 CrossRef CAS PubMed.
- Z. Ning, D. Zhitomirsky, V. Adinolfi, B. Sutherland, J. Xu, O. Voznyy, P. Maraghechi, X. Lan, S. Hoogland and Y. Ren,
et al.
, Adv. Mater., 2013, 25, 1719–1723 CrossRef CAS PubMed.
- E. T. McClure, M. R. Ball, W. Windl and P. M. Woodward, Chem. Mater., 2016, 28, 1348–1354 CrossRef CAS.
- M. R. Filip, S. Hillman, A. A. Haghighirad, H. J. Snaith and F. Giustino, J. Phys. Chem. Lett., 2016, 7, 2579–2585 CrossRef CAS PubMed.
- F. Wei, Z. Deng, S. Sun, F. Xie, G. Kieslich, D. M. Evans, M. A. Carpenter, P. D. Bristowe and A. K. Cheetham, Mater. Horiz., 2016, 3, 328–332 RSC.
- Z. Deng, F. Wei, S. Sun, G. Kieslich, A. K. Cheetham and P. D. Bristowe, J. Mater. Chem. A, 2016, 4, 12025–12029 CAS.
- Y.-Y. Sun, J. Shi, J. Lian, W. Gao, M. L. Agiorgousis, P. Zhang and S. Zhang, Nanoscale, 2016, 8, 6284–6289 RSC.
- S. Wang, D. B. Mitzi, G. A. Landrum, H. Genin and R. Hoffmann, J. Am. Chem. Soc., 1997, 119, 724–732 CrossRef CAS.
- D. B. Mitzi, Inorg. Chem., 1996, 35, 7614–7619 CrossRef CAS.
- D. B. Mitzi and P. Brock, Inorg. Chem., 2001, 40, 2096–2104 CrossRef CAS PubMed.
- K. R. Maxcy, R. D. Willett, D. B. Mitzi and A. Afzali, Acta Crystallogr., Sect. E: Struct. Rep. Online, 2003, 59, m364–m366 CAS.
- W.-X. Chai, J. Lin, L. Song, L.-S. Qin, H.-S. Shi, J.-Y. Guo and K.-Y. Shu, Solid State Sci., 2012, 14, 1226–1232 CrossRef CAS.
- S. A. Adonin, I. D. Gorokh, D. G. Samsonenko, M. N. Sokolov and V. P. Fedin, Chem. Commun., 2016, 52, 5061–5063 RSC.
- S. Sun, S. Tominaka, J.-H. Lee, F. Xie, P. D. Bristowe and A. K. Cheetham, APL Mater., 2016, 4, 031101 CrossRef.
- N. A. Yelovik, A. V. Mironov, M. A. Bykov, A. N. Kuznetsov, A. V. Grigorieva, Z. Wei, E. V. Dikarev and A. V. Shevelkov, Inorg. Chem., 2016, 55, 4132–4140 CrossRef CAS PubMed.
- C. Silvestru, H. J. Breunig and H. Althaus, Chem. Rev., 1999, 99, 3277–3328 CrossRef CAS PubMed.
-
D. B. Mitzi, in Synthesis, Structure, and Properties of Organic-Inorganic Perovskites and Related Materials, ed. K. D. Karlin, John Wiley & Sons, Inc., Hoboken, NJ, USA, 2007, vol. 48, pp. 1–121 Search PubMed.
- M. A. Green, K. Emery, Y. Hishikawa, W. Warta and E. D. Dunlop, Prog. Photovolt: Res. Appl., 2016, 24, 905–913 CrossRef.
Footnote |
† These authors contributed equally to this work. |
|
This journal is © The Royal Society of Chemistry 2017 |
Click here to see how this site uses Cookies. View our privacy policy here.