DOI:
10.1039/C5SC04673D
(Edge Article)
Chem. Sci., 2016,
7, 3240-3247
Dynamic helical cyclophanes with two quadruply-bridged planes arranged in an “obverse and/or reverse” relation†
Received
4th December 2015
, Accepted 29th January 2016
First published on 29th January 2016
Abstract
We describe the design of two types of cyclophanes that generate dynamic helicity through the twisting of two planes in a clockwise or counterclockwise direction to give (M)- or (P)-helicity. We used a rectangular and anisotropic plane of 1,2,4,5-tetrakis(phenylethynyl)benzene (TPEB), since it can be stacked in pairs in two ways, in parallel or orthogonally, to be identified as distinct cyclophane molecules. We adopted a synthetic strategy for obtaining these two cyclophanes as a mixture using a macrocyclic intermediate that possessed two rotatable phenyl rings. We introduced necessary parts into the rotators to give a mixture of rotational isomers leading to a parallel or orthogonal arrangement of TPEBs, and then doubly bridged two planes of TPEB to form quadruply-bridged cyclophanes. We consider that such two planes in each cyclophane are in an “obverse and/or reverse” relation. In each cyclophane, we found unique dynamic helical forms with (M)- or (P)-helicity as well as an inherently non-chiral form. Normally, the screw-sense preference of dynamic helicity would be controlled through the intramolecular or supramolecular transmission of central chirality, when a chiral auxiliary is attached to the cyclophanes or a chiral guest is allowed to form a complex with the cyclophanes. In a case where two different substitution groups were used on bridging units to generate planar chirality in each cyclophane, the screw-sense preference was controlled through the arrangement of these substitution groups, and did not depend on the transmission of central chirality. Two different substitution groups desymmetrize the enantiomeric forms with (M)- or (P)-helicity generated in each dynamic helical cyclophane so that two dynamic helical forms with (M)- or (P)-helicity can be in a diastereomeric relation. Thus, a particular screw sense of dynamic helicity can be preferred, regardless of whether or not the two substitution groups possess some chiral element.
Introduction
Helical twisting of achiral components that are stacked in a columnar assembly is a well-known method for creating helical architectures.1–7 The preference for a particular screw sense in the assembly is induced by the transmission of central chirality that exists in the periphery of an achiral component, or in external chiral additives. This methodology for generating and controlling helical chirality is suitable for layered structures in a molecule.8 In the case of supramolecular assemblies or in molecules with a layered structure, linear,2,8a,b,9a trigonal,3,8c,d,9b–e tetragonal,4,8e–g,9f hexagonal5 or more highly symmetric6 molecule(s) have often been used as an achiral component, and such regular polygonal symmetry would appear to provide a single manner of helical stacking. We were interested in a rectangular and anisotropic shape of 1,2,4,5-tetrasubstituted benzene, since it can be stacked in two distinct manners (Scheme 1).10 However, these two states might merge to be identical during twisting if the two planes are not fixed in relation to each other. Bridging of these two planes with multiple covalent bonds would enable the two states to be individualized as distinct cyclophane molecules.10a,11 We designed two types of cyclophanes A and B with two planes of 1,2,4,5-tetrakis(phenylethynyl)benzene (TPEB) that are stacked in pairs (Fig. 1). We used terephthalamide as a four-fold bridge in both types of cyclophanes. We synthesized the two types of cyclophanes A and B as a single mixture, and separated them by HPLC. As mentioned above, two planes are arranged in parallel (A) or orthogonally (B) (Scheme 1). To realize this relation in a cyclophane scaffold, we assumed an imaginary cyclophane with a two-fold bridge, in which one of the two diametrical axes is bridged with another on the upper and lower planes (Scheme 2). Rotation of a particular plane about the doubly bridged diametrical axis leads to isomerization between A and B, independent of whether such rotation is actually allowed or not. Double-rotation of the two planes leads to isomerization between the two enantiomeric forms of type B. If we consider this “obverse and/or reverse” relation between two planes, we can use a macrocyclic intermediate that possesses two rotatable phenyl rings. We introduced necessary parts into the rotators to give a mixture of rotational isomers, and then doubly bridged two planes to form quadruply-bridged cyclophanes A and B as a mixture (Scheme 2). The product ratio of these two cyclophanes should not significantly depend on the final ring-closing reaction even though their potential energies are different, but rather should depend on an earlier stage where at least one rotator is expected to be rotatable until the last part has been introduced.
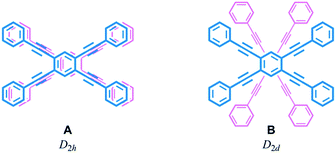 |
| Scheme 1 Two types of stacking of anisotropic planes (1,2,4,5-tetrasubstituted benzene). | |
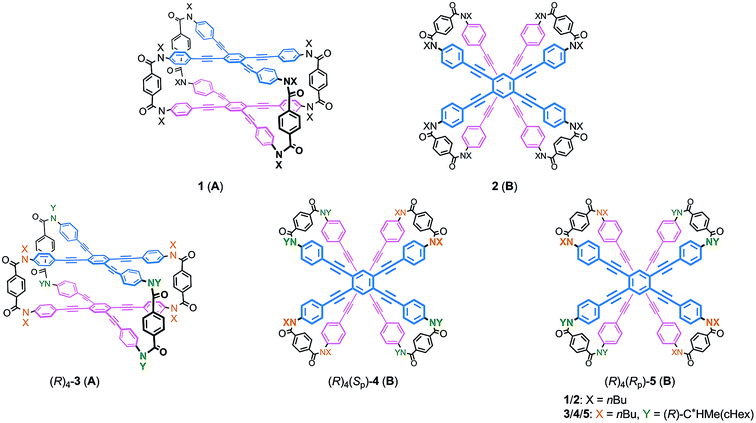 |
| Fig. 1 Chemical structures of dynamic helical cyclophanes 1-5 with two quadruply-bridged planes. | |
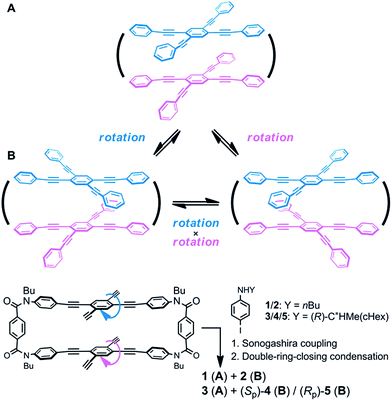 |
| Scheme 2 The “obverse and/or reverse” relation in two cyclophanes A and B, and a synthetic strategy for obtaining both quadruply-bridged cyclophanes A and B. | |
We envisioned that the helical twisting of two planes in each covalently bridged cyclophane could create unique dynamic helicity (Scheme 3). We designate the conformations of these cyclophanes as M4-A, M2P2-B and so on, where M and P denote the partial helicity that is generated between two bridged phenylethynyl groups. In cyclophane A, two planes would twist to create in concert four-fold partial helicity aligned in the same direction toward M (M4-A) or P (P4-A) (Scheme 3a). In cyclophane B, two planes are arranged orthogonally and thus are inherently twisted so that they can be considered a meso-like form (M2P2-B). Additional twisting is allowed by the inversion of partial helicity only at a particular two-fold bridge across the central benzene rings of TPEBs, and would lead to dynamic helical forms M4-B or P4-B (Scheme 3b). Note that M4-A and M4-B are different molecules, although they have been assigned the same symbol. Normally, the screw-sense preference of such dynamic helicity would be controlled through a transmission of central chirality,8b,c,12 as mentioned above.
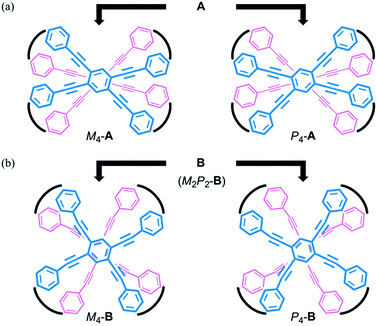 |
| Scheme 3 Generation of dynamic helicity through helical twisting of two planes in cyclophanes (a) A and (b) B with a four-fold bridge. | |
Recently, we reported the design of a planar chiral cyclophane of type A through the differentiation of substitution groups X and Y (X ≠ Y) on each amide nitrogen of all four bridging units, and the control of screw-sense preference of dynamic helicity that was independent of any transmission of chirality.13 Here we demonstrate an alternative design with respect to type B (Scheme 4). We again used two different substitution groups X and Y (X ≠ Y). Two pairs of X are arranged at one bridge and another across the central benzene rings of TPEBs, and two pairs of Y are similarly arranged in the remaining two bridges. In cyclophanes of type B, the arrangement of X and Y generates planar chirality14 [(Sp)-4 (B) and (Rp)-5 (B)]. Such a planar chiral cyclophane is assured to be configurationally stable during dynamic interconversions among conformations. In an inherently twisted but meso-like form (M2P2-B), X is on a bridge with partial M-helicity and Y is on a bridge with partial P-helicity. The molecule is only allowed to deform once by inversion of the original partial helicity at a particular two-fold bridge of the four bridges. Deformation at two bridges with an X group would lead to the generation of a dynamic helical form with global (P)-helicity (P4-B). Another dynamic helical form (M4-B) with the contrary sense would be generated due to deformation at two bridges with a Y group. These two dynamic helical forms M4-B and P4-B are diastereomeric (X ≠ Y) and energetically nonequivalent. Thus, a particular screw sense of dynamic helicity would be preferred through the arrangement of X and Y. In a mirrored isomer with planar chirality, a contrary preference would be created by arrangement of the identical pair of X and Y (X ≠ Y).
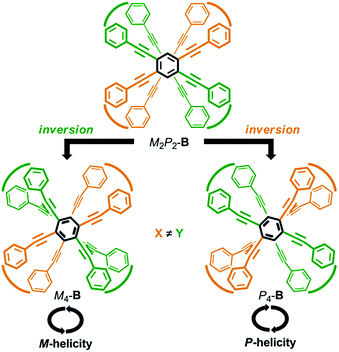 |
| Scheme 4 Generation of planar chirality and control of screw-sense preference of dynamic helicity through the arrangement of X and Y (X ≠ Y) on the bridging units (terephthalamide), which are drawn as an arc for clarity. Only a particular planar chiral enantiomer is depicted. If we assume that Y is the higher priority group, the stereochemistry of the depicted cyclophane (B) is Sp. | |
Results and discussion
Synthesis of cyclophanes with two planes arranged in an “obverse and/or reverse” relation
We obtained the cyclophanes 1 (A) and 2 (B) in pure form (1/2 = 55
:
45) by HPLC separation (Schemes 2 and S1†). Due to the presence of mirrors in both 1 (D2h) and 2 (D2d), they are not chiral and were used to investigate the supramolecular transmission of central chirality upon complexation with a chiral guest. Alternatively, there is no mirror in the cyclophanes (R)4-3, (R)4-4 and (R)4-5, due to the presence of central chirality (R) in the Y group. (R)4-3 (A) is chiral but does not possess a chiral plane, and therefore it was used to investigate the intramolecular transmission of central chirality associated with the cyclophane. In cyclophanes of type B, planar chirality is inherently generated through the arrangement of X and Y (X ≠ Y). Only the differentiation and arrangement of X and Y are essential for producing planar chirality. Central chirality (R) in the Y group is not involved in the generation of planar chirality. A diastereomeric mixture of (R)4-3, (R)4-4 and (R)4-5 in a ratio of 62
:
25
:
13 was separated in this order by HPLC to give (R)4-3 and (R)4-4 in pure form, and (R)4-5 as a mixture containing less than 6% (R)4-4. We did not determine the absolute configuration (Sp) or (Rp) with regard to the planar chirality of 4 and 5, but arbitrarily assigned the second and third fractions to (Sp)-4 and (Rp)-5, respectively, to describe the following results. As a chiral guest, we used diammonium salts8c (S)2-6 and (R)2-6 to investigate the supramolecular transmission of central chirality during complexation. As references, we prepared a single-layer TPEB derivative (R)2-7, and a doubly-bridged cyclophane (R)4-8 (Fig. 2).
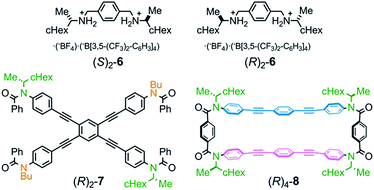 |
| Fig. 2 Chemical structures of chiral ditopic guests (S)2-6/(R)2-6, and references (R)2-7 and (R)4-8. | |
Molecular structures of cyclophanes
A conformational search for a model 2′ (B) [X = Me] predicted that an inherently twisted but meso-like form (M2P2-2′) was the most energy-minimized structure (Fig. 3a), similar to that seen in a crystal.15 In addition, a global helical form M4-2′ was also found at a higher energy level (+22.8 kJ mol−1) (Fig. 3b). Either form of 2′ was predicted to exist at higher energies than M4-1′ (−58.1 kJ mol−1 relative to the most minimized potential energy for M2P2-2′).
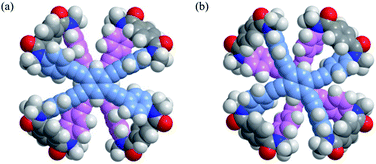 |
| Fig. 3 Energy-minimized structures for a model 2′ (B) [NMe], (a) M2P2-2′ (rel. 0 kJ mol−1) and (b) M4-2′ (+22.8 kJ mol−1), obtained by a conformational search using MacroModel software (v9.9 OPLS_2005, Monte Carlo Multiple Minimum method, non-solvated, 50 000 steps). Only a particular enantiomeric form with (M)-helicity is depicted. | |
Next, we investigated the dynamic structure in solution by NMR spectroscopy (Fig. S2†). The 1H NMR spectra of both 1 (A) and 2 (B) showed a single set of averaged resonances at room temperature (Fig. S2a†). The aromatic protons HA on the central benzene ring of TPEB in 1 and 2 with two planes appeared more upfield compared to that in single-layer 7, which might be characteristic of these two cyclophanes. The chemical shifts for HB and HD on the peripheral phenylethynyl blade of TPEB in 1 and 2 were close to those in macrocyclic 8 rather than those in 7. This similarity indicated that macrocyclic 8 represented a substructure of these cyclophanes better than 7. Energy-minimized structures for a model 8′ [NMe] are summarized in Fig. S3.† Since the chemical shifts for the averaged resonances in the spectra of 1 and 2 changed with temperature (Fig. S4a†), conformations with different structures underwent dynamic interconversions in each solution.16
The 1H NMR spectrum of (R)4-3 (A) showed a single set of averaged resonances that included two differentiated singlet peaks for HA and HA′, which indicated that global helical forms M4-A and P4-A, rather than an eclipsed form, predominated in solution, and interconverted to each other on the NMR timescale. Helical twisting of two planes in cyclophane A creates two non-equivalent spaces with different dimensions, where one is narrower than the other (Scheme 3a). In fact, several pairs on each of the upper and lower planes were differentiated (Fig. S2a†). Such differentiation due to a conformational preference for dynamic helical forms was also supported by 13C NMR (Fig. S2b†). If we consider that an eclipsed form is dominant, the two planes should be equivalent and should show a spectral pattern similar to that of single-layer 7.
The 1H NMR spectra of both (R)4-4 (B) and (R)4-5 (B) showed a single set of averaged resonances with a spectral pattern similar to that of 7, which could be explained with either form M4-B, M2P2-B or P4-B, and indicated that these diastereomeric forms undergo dynamic interconversions in solution.
Control of screw-sense preference of dynamic helicity through the intramolecular transmission of central chirality (R) in (R)4-3 (A), and through the arrangement of two different substitution groups in (R)4(Sp)-4 and (R)4(Rp)-5
The UV-vis spectrum of a cyclophane (R)4-3 (A) showed an absorption maximum [λmax/nm (log
ε) 315 (5.15)] and a shoulder at a longer wavelength region (Fig. 4A, left), which seemed to be characteristic of TPEB,17 although they were hypsochromically shifted and the intensity was markedly attenuated throughout the absorption region, compared to the spectrum of single-layer TPEB 7 [332 (5.11) and sh. 375 (4.74)]. These spectral perturbations seen for 3 might be attributed to the reduction of coplanarity due to the local twisting of peripheral phenylethynyl blades with respect to the central benzene ring of TPEB. Instead, we found a similar appearance in the spectrum of macrocyclic 8 [309 (4.97)], which is composed of two chromophores of 1,4-bis(phenylethynyl)benzene, bridged by a two-fold terephthalamide, and such chromophores might be present as an effective conjugation even in 3. In the CD spectrum of (R)4-3 (A), we found compositive Cotton effects in the absorption region of 3 (Fig. 4A, right). On the other hand, in the spectrum of (R)2-7, small negatively signed Cotton effects were present throughout the absorption region of 7. These Cotton effects of (R)2-7 were completely different from those of (R)4-3 (A), and were considered to have no relation with any helical structure, but rather originated from the local chiral environment around the central chiral auxiliary. Again, we found negatively signed Cotton effects in the spectrum of (R)4-8, although it was predicted to adopt helical forms. We considered that these negatively signed Cotton effects of (R)4-8 could also be attributed to the local chiral environment, and that the intramolecular transmission of chirality would not be valid in a case where the two amide carbonyls in the bridging unit adopted a locally non-helical form (Fig. S3†).18 The Cotton effects seen for (R)4-3 (A) could not be explained at all by assuming an eclipsed form or a local chiral environment around the chiral auxiliary, but could be explained by an induced preference for a particular screw sense of dynamic helicity through the intramolecular transmission of central chirality. At least one of the two chiral auxiliaries on the bridging unit should always be placed in a narrower space that is created by the helical twisting of the two planes in the cyclophane, and can act as a directing group to prefer a particular screw sense of dynamic helicity. We confirmed that the Cotton effects were enhanced with a decrease in temperature and attenuated with an increase in temperature (Fig. S6a†). This result indicated that the two diastereomeric forms with global (M)- or (P)-helicity undergo dynamic interconversion in solution.
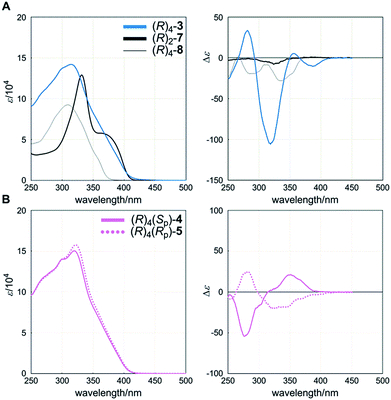 |
| Fig. 4 UV (left) and CD (right) spectra of (A) (R)4-3, (R)2-7 and (R)4-8, and (B) (R)4(Sp)-4 and (R)4(Rp)-5. All spectra were measured in dichloromethane at room temperature. | |
Cyclophanes (R)4(Sp)-4 (B) and (R)4(Rp)-5 (B) showed absorption [λmax/nm (log
ε) 320 (5.18) for 4, and 322 (5.20) for 5] similar in appearance to that of (R)4-3 (A) (Fig. 4B, left). In these absorption regions, we found compositive and global bisignated Cotton effects in the CD spectra of each planar chiral cyclophane (Fig. 4B, right). Notably, the two spectra were pseudo-mirrored. These Cotton effects should be attributed to dynamic helical forms, rather than to the local chiral environment, since the identical chiral auxiliary was present in both cyclophanes. We considered that it may be difficult for a meso-like form to produce Cotton effects due to the intramolecular cancellation of partial helicity, even though it would be the most common form in solution. If the central chiral auxiliary preferred a particular screw sense of dynamic helicity through intramolecular transmission, it should be manifested because both dynamic helical forms M4-B and P4-B were provided in each planar chiral isomer (Scheme 4).19 If this assumption is valid, then similarly signed Cotton effects, not pseudo-mirrored, should appear in each spectrum. Thus, we considered that these Cotton effects showed an induced preference for a particular screw sense of dynamic helicity through the arrangement of two different substitution groups X and Y. VT CD measurements supported the contribution of diastereomeric forms with (M)- or (P)-helicity that dynamically interconverted in solution to the creation of pseudo-mirrored Cotton effects (Fig. S6b and c†).
In the following section, we confirmed the presence of dynamic helical forms that were unique to each type of cyclophane A and B using simple scaffolds 1 (A) and 2 (B), which do not possess any chiral element other than dynamic helicity (Scheme 3).
Control of screw-sense preference of dynamic helicity through the supramolecular transmission of central chirality (S,S) or (R,R) in the guest to dynamic helicity of achiral cyclophane hosts 1 (A) and 2 (B)
Two amide carbonyls in the bridging unit are allowed to twist helically in a conrotatory (m- or p-helicity) or disrotatory manner (non-helicity) around a local C2 axis. Thus, we considered that helical twisting of the two amide carbonyls would provide local dynamic helicity in the bridge (Fig. 5). We envisioned that the control of local dynamic helicity would lead to global control of the preference for a dynamic helical form M4- or P4- in these cyclophanes [1 (A) and 2 (B)]. The direction of the local twisting of the two amide carbonyls would be controlled by the supramolecular transmission of central chirality, when a chiral ditopic guest is captured at the two amide carbonyls.
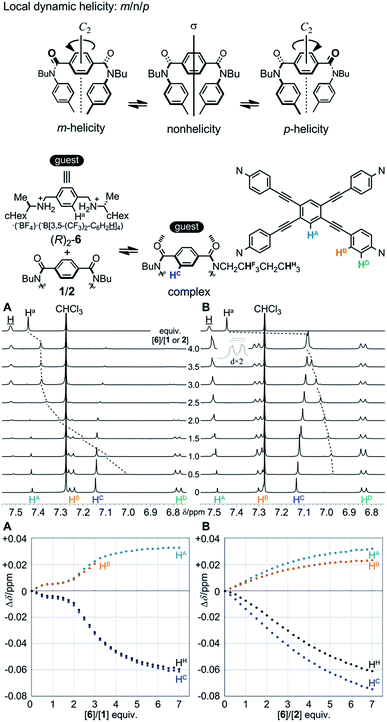 |
| Fig. 5 Partial 1H NMR (400 MHz) spectra of 1 (A) (0.5 mM)/2 (B) (0.5 mM) in the presence of (R)2-6 [0 (host only)-4 equiv.] and the spectrum of (R)2-6 (top), and titration curves for the complexation of 1 (A)/2 (B) with (R)2-6. All spectra were measured in 2 vol% acetonitrile-d3/chloroform-d at 298 K. | |
We first investigated the complexation of cyclophane 1 (A) with a chiral ditopic guest (R)2-6 by 1H NMR spectroscopy, measured in chloroform-d containing 2 vol% acetonitrile-d3 at 298 K (Fig. 5A). When the host and guest were mixed, we found complexation-induced shifts for both phenylene protons HC and Ha, associated with 1 and 6, respectively, which indicated that the guest was captured at the two amide carbonyls of a bridging unit through the formation of double hydrogen bonds. Through a titration experiment, we obtained complicated discontinuous titration curves that included several inflection points, especially at around the addition of two equivalents of 6. In an early stage, we could confirm that the guest was mostly in a complexed state through ditopic binding, as shown by a large upfield shift for Ha, which was later insignificant upon the further addition of 6. For these inflection points, we could not analyze the complexation quantitatively. Although signals were broadened in a later stage, we tried to trace changes in the chemical shift for other protons far from the binding site, which indicated that complexation induced some change in the conformation of 1.
Next, we investigated the complexation of 2 (B) with (R)2-6 under similar conditions (Fig. 5B). Similar to the above case, we confirmed complexation-induced shifts for both phenylene protons HC and Ha, which indicated the formation of double hydrogen bonds at the two amide carbonyls. Unlike the above case, we fortunately obtained titration curves that showed a sigmoidal curve, which indicated that we could analyze complexation as a type of positive allosteric binding.8e–g,20 In a later stage, we found that aromatic protons HB (and HD) on TPEB in 2 (B) were differentiated, as seen for 4 (B) or 5 (B). Although no conformation of the host is homotopic (Scheme 3b), we tried to analyze the complexation by a Job plot and Hill plot (Fig. S8†).21 In Job plots, we could find a maximum or minimum for several protons throughout the host molecule at 0.2 < χ2 < 0.3, and for protons in the guest at 0.7 < χ6 < 0.8 (Fig. S8a†). We estimated that the binding constant Ka was 108 to 109 M−4 and the Hill coefficient was 2.5–2.7 on the basis of Hill plots for several protons (Fig. S8b†), if we assumed that a 1
:
4 complex was formed.
The UV-vis spectrum of 1 (A) showed an absorption maximum at 318 nm and a shoulder band at around 360 nm. When we added (R)2-6 to a solution of 1 in dichloromethane at room temperature, the former band increased with a slight bathochromic shift and the latter band increased (Fig. 6A, upper), which seemed to change toward the spectrum of 7. In an early stage, small but significant Cotton effects similar to those in the spectrum of (R)4-3 (A) were induced in the absorption region of 1 (Fig. 6A, lower). Addition of the antipodal guest (S)2-6 induced mirror-imaged Cotton effects. These results indicated that a particular screw sense of dynamic helicity was preferred in a complex, at least in an early stage. These Cotton effects were attenuated and ultimately disappeared upon further addition of the guest. We considered that these spectral changes resulted from the cyclophane host 1 undergoing a change in conformation from dynamic helical forms to a less- or non-helical form during complexation.
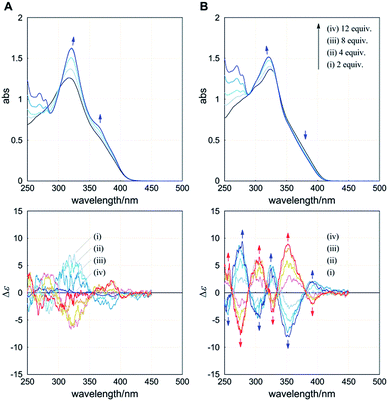 |
| Fig. 6 UV (upper) and CD (lower) spectra of 1 (A) (7.5 × 10−5 M) and 2 (B) (9.9 × 10−5 M) in the presence of (R)2-6 (blue lines) or (S)2-6 (red lines) [0 (black, host only), 2, 4, 8 and 12 equiv.]. All spectra were measured in dichloromethane at 293 K. | |
The UV-vis spectrum of 2 (B) showed an absorption maximum at 324 nm and a shoulder band at a longer wavelength (Fig. 6B, upper). When we added (R)2-6 to a solution of 2 under conditions similar to those in the above case for 1, the former band increased with a slight hypsochromic shift and the latter band decreased. In the CD spectrum of 2, we found a gradual increase in the Cotton effects to show several compositive couplets upon gradual addition of the guest (Fig. 6B, lower). When the antipodal guest (S)2-6 was added, completely mirror-imaged Cotton effects were induced. These spectral changes indicated a complexation-induced change in conformation from an inherently twisted but meso-like form M2P2 to dynamic helical forms M4 or P4, and the cyclophane host preferred a particular screw sense of dynamic helicity generated in a complex through the supramolecular transmission of central chirality in the guest.
Conclusions
We have demonstrated the design and control of screw-sense preference of unique dynamic helicity that was generated by the twisting of two planes in a cyclophane (dynamic helical cyclophane). We used a rectangular and anisotropic plane that was stacked in parallel or orthogonally in pairs in two distinct cyclophanes with D2h (A) or D2d (B) symmetry. We considered that such two planes in each cyclophane are in an “obverse and/or reverse” relation. The arrangement of two different substitution groups X and Y (X ≠ Y) on bridges generates planar chirality in a particular cyclophane of type A13 or B. These planar chiral cyclophanes are configurationally stable and conformationally dynamic. We confirmed that the two different substitution groups can act as directing groups to control the screw-sense preference of unique dynamic helicity in each planar chiral cyclophane through the arrangement of two different substitutions, independent of any transmission of chirality.
Notes and references
- C. C. Lee, C. Grenier, E. W. Meijer and A. P. H. J. Schenning, Chem. Soc. Rev., 2009, 38, 671 RSC; D. Pijper and B. L. Feringa, Soft Matter, 2008, 4, 1349 RSC; A. R. A. Palmans and E. W. Meijer, Angew. Chem., Int. Ed., 2007, 46, 8948 CrossRef CAS PubMed.
- S. Kawano, N. Fujita and S. Shinkai, Chem.–Eur. J., 2005, 11, 4735 CrossRef CAS PubMed; J.-H. Ryu, H.-J. Kim, Z. Huang, E. Lee and M. Lee, Angew. Chem., Int. Ed., 2006, 45, 5304 CrossRef PubMed; S. J. George, Ž. Tomović, M. M. J. Smulders, T. F. A. de Greef, P. E. L. G. Leclère, E. W. Meijer and A. P. H. J. Schenning, Angew. Chem., Int. Ed., 2007, 46, 8206 CrossRef PubMed; Y. Li, G. Li, X. Wang, W. Li, Z. Su, Y. Zhang and Y. Ju, Chem.–Eur. J., 2009, 15, 6399 CrossRef PubMed; C. Roche, H.-J. Sun, P. Leowanawat, F. Araoka, B. E. Partridge, M. Peterca, D. A. Wilson, M. E. Prendergast, P. A. Heiney, R. Graf, H. W. Spiess, X. Zeng, G. Ungar and V. Percec, Nat. Chem., 2016, 8, 80 CrossRef PubMed.
- M. L. Bushey, T.-Q. Nguyen, W. Zhang, D. Horoszewski and C. Nuckolls, Angew. Chem., Int. Ed., 2004, 43, 5446 CrossRef CAS PubMed; T. Ishi-i, R. Kuwahara, A. Takata, Y. Jeong, K. Sakurai and S. Mataka, Chem.–Eur. J., 2006, 12, 763 CrossRef PubMed; C. Bao, M. Jin, R. Lu, Z. Song, X. Yang, D. Song, T. Xu, G. Liu and Y. Zhao, Tetrahedron, 2007, 63, 7443 CrossRef; F. García, P. M. Viruela, E. Matesanz, E. Ortí and L. Sánchez, Chem.–Eur. J., 2011, 17, 7755 CrossRef PubMed; N. Veling, R. van Hameren, A. M. van Buul, A. E. Rowan, R. J. M. Nolte and J. A. A. W. Elemans, Chem. Commun., 2012, 48, 4371 RSC; F. García and L. Sánchez, J. Am. Chem. Soc., 2012, 134, 734 CrossRef PubMed.
- L. Liu, Y. Li and M. Liu, J. Phys. Chem. C, 2008, 112, 4861 Search PubMed; Y. Zhang, P. Chen and M. Liu, Chem.–Eur. J., 2008, 14, 1793 CrossRef CAS PubMed.
- J. Li, W. Zhou, J. Yang, X. Lang and P. Huang, J. Colloid Interface Sci., 2013, 395, 99 CrossRef CAS PubMed; X. Zhou, G. Liu, K. Yamato, Y. Shen, R. Cheng, X. Wei, W. Bai, Y. Gao, H. Li, Y. Liu, F. Liu, D. M. Czajkowsky, J. Wang, M. J. Dabney, Z. Cai, J. Hu, F. V. Bright, L. He, X. C. Zeng, Z. Shao and B. Gong, Nat. Commun., 2012, 3, 949 CrossRef PubMed; H.-J. Kim, S.-K. Kang, Y.-K. Lee, C. Seok, J.-K. Lee, W.-C. Zin and M. Lee, Angew. Chem., Int. Ed., 2010, 49, 8471 CrossRef PubMed; K. Nakamura, H. Okubo and M. Yamaguchi, Org. Lett., 2001, 3, 1097 CrossRef PubMed.
- H. Yamagishi, T. Fukino, D. Hashizume, T. Mori, Y. Inoue, T. Hikima, M. Takata and T. Aida, J. Am. Chem. Soc., 2015, 137, 7628 CrossRef CAS PubMed.
- K. Sakajiri, T. Hirama, K. Yasuda, S. Kutsumizu and J. Watanabe, Bull. Chem. Soc. Jpn., 2013, 86, 940 CrossRef CAS; F. García, F. Aparicio, M. Marenchino, R. Campos-Olivas and L. Sánchez, Org. Lett., 2010, 12, 4264 CrossRef PubMed.
-
(a) Y. Wu, M. Frasconi, D. M. Gardner, P. R. McGonigal, S. T. Schneebeli, M. R. Wasielewski and J. F. Stoddart, Angew. Chem., Int. Ed., 2014, 53, 9476 CrossRef CAS PubMed;
(b) R. Katoono, H. Kawai, K. Fujiwara and T. Suzuki, Chem. Commun., 2005, 5154 RSC;
(c) R. Katoono, K. Fujiwara and T. Suzuki, Chem. Commun., 2014, 50, 5438 RSC;
(d) P. Rajakumar and M. Srisailas, Tetrahedron Lett., 2002, 43, 1909 CrossRef CAS;
(e) M. Takeuchi, T. Imada and S. Shinkai, Angew. Chem., Int. Ed., 1998, 37, 2096 CrossRef CAS;
(f) A. Sugasaki, M. Ikeda, M. Takeuchi, A. Robertson and S. Shinkai, J. Chem. Soc., Perkin Trans. 1, 1999, 3259 RSC;
(g) A. Sugasaki, M. Ikeda, M. Takeuchi, K. Koumoto and S. Shinkai, Tetrahedron, 2000, 56, 4717 CrossRef CAS.
-
(a) K. Katagiri, T. Tohaya, H. Masu, M. Tominaga and I. Azumaya, J. Org. Chem., 2009, 74, 2804 CrossRef CAS PubMed;
(b) Y. Rubin, T. C. Parker, S. I. Khan, C. L. Holliman and S. W. McElvany, J. Am. Chem. Soc., 1996, 118, 5308 CrossRef CAS;
(c) M. Tominaga, H. Masu, K. Katagiri and I. Azumaya, Tetrahedron Lett., 2007, 48, 4369 CrossRef CAS;
(d) H. Lee, T. H. Noh and O.-S. Jung, Angew. Chem., Int. Ed., 2013, 52, 11790 CrossRef CAS PubMed;
(e) H. Y. Lee, J. Park, M. S. Lah and J.-I. Hong, Chem. Commun., 2007, 5013 RSC;
(f) C. Zhang, Q. Wang, H. Long and W. Zhang, J. Am. Chem. Soc., 2011, 133, 20995 CrossRef CAS PubMed.
-
(a) F. Vögtle and N. Wester, Liebigs Ann. Chem., 1978, 545 CrossRef;
(b) X. Feng, W. Pisula and K. Müllen, J. Am. Chem. Soc., 2007, 129, 14116 CrossRef CAS PubMed.
- H. Hopf, Tetrahedron, 2008, 64, 11504 CrossRef CAS; H. Hinrichs, A. J. Boydston, P. G. Jones, K. Hess, R. Herges, M. M. Haley and H. Hopf, Chem.–Eur. J., 2006, 12, 7103 CrossRef PubMed; Y. Morisaki, M. Gon, T. Sasamori, N. Tokitoh and Y. Chujo, J. Am. Chem. Soc., 2014, 136, 3350 CrossRef PubMed; T. Furo, T. Mori, T. Wada and Y. Inoue, J. Am. Chem. Soc., 2005, 127, 8242 CrossRef PubMed.
- We have been interested in the control of screw-sense preference of dynamic helicity based on terephthalamide macrocycles through the supramolecular and/or intramolecular transmission(s) of central chirality, as reported in the following papers; R. Katoono, S. Kawai, K. Fujiwara and T. Suzuki, Chem. Sci., 2015, 6, 6592 RSC; R. Katoono, Y. Tanaka, K. Kusaka, K. Fujiwara and T. Suzuki, J. Org. Chem., 2015, 80, 7613 CrossRef CAS PubMed; R. Katoono, Y. Tanaka, K. Fujiwara and T. Suzuki, J. Org. Chem., 2014, 79, 10218 CrossRef PubMed; R. Katoono, K. Kusaka, K. Fujiwara and T. Suzuki, Chem.–Asian J., 2014, 9, 3182 CrossRef PubMed; R. Katoono, H. Kawai, M. Ohkita, K. Fujiwara and T. Suzuki, Chem. Commun., 2013, 49, 10352 RSC.
- R. Katoono and T. Suzuki, Chem. Commun., 2016, 52, 1029 RSC.
- S. E. Gibson and J. D. Knight, Org. Biomol. Chem., 2003, 1, 1256 Search PubMed; T. Mori and Y. Inoue, Top. Curr. Chem., 2011, 298, 99 CrossRef CAS PubMed.
- A single-crystal X-ray analysis of cyclophane 2 (B) [X = nBu] revealed that the two planes were arranged orthogonally to adopt a meso-like form denoted by M2P2-2 (Fig. S1†). Regarding type A,13 we reported that the two planes in cyclophane 1 (A) [X = nBu] were forced to adopt an eclipsed form with a slight deformation of the planes to infill the cavity. A conformational search for a model 1′ (A) [X = Me] predicted that a helically twisted form (M4-1′) was the most energy-minimized structure.
- Even though the temperature was lowered to 223 K, there was no sign of decoalescence due to faster dynamic interconversions among conformations than the NMR timescale.
- K. Kondo, S. Yasuda, T. Sakaguchi and M. Miya, J. Chem. Soc., Chem. Commun., 1995, 55 RSC; J. A. Marsden, J. J. Miller, L. D. Shirtcliff and M. M. Haley, J. Am. Chem. Soc., 2005, 127, 2464 CrossRef CAS PubMed.
- Indeed, we found bisignated Cotton effects induced in the CD spectrum of (R)4-8 in the presence of a chiral ditopic guest (R)2-6 (Fig. S5†). The complexation-induced Cotton effects, as well as an induced change in absorption in the UV spectrum, showed that the host underwent a change in conformation from a locally non-helical form to locally dynamic helical forms upon complexation, and a particular screw sense of global dynamic helicity was preferred in a complexed state through a transmission of central chirality in the host and/or guest.
- The central chiral auxiliary might have acted as a directing group to prefer a particular screw sense of dynamic helicity through intramolecular transmission of the central chirality. In some cases, e.g., in a complexed state, it can contribute to determining a local conformation of the host, as shown by the following examples. The Cotton effects obtained from (R)4(Sp)-4 (B) were distinctly modulated upon diastereomeric complexation with (R)2-6 or (S)2-6 (Fig. S7a†). These results indicated that the host underwent some change in conformation in response to central chirality in each guest through the supramolecular transmission of chirality. Alternatively, the Cotton effects obtained from (R)4(Rp)-5 (B) were similarly modulated during diastereomeric complexation with (R)2-6 or (S)2-6 (Fig. S7b†). These results indicated that the host could adopt an identical conformation in either diastereomeric complex through the intramolecular transmission of central chirality associated with the host.
- J. S. Park, F. L. Derf, C. M. Bejger, V. M. Lynch, J. L. Sessler, K. A. Nielsen, C. Johnsen and J. O. Jeppesen, Chem.–Eur. J., 2010, 16, 848 CrossRef CAS PubMed; J. Setsune and K. Watanabe, J. Am. Chem. Soc., 2008, 130, 2404 CrossRef PubMed; R. Sakai, S. Okade, E. B. Barasa, R. Kakuchi, M. Ziabka, S. Umeda, K. Tsuda, T. Satoh and T. Kakuchi, Macromolecules, 2010, 43, 7406 CrossRef.
- A. Cornish-Bowden and D. E. Koshland Jr, J. Mol. Biol., 1975, 95, 201 CrossRef CAS PubMed.
Footnote |
† Electronic supplementary information (ESI) available: X-ray and energy-minimized structures, NMR and CD spectroscopic data, and experimental details of new compound preparation. CCDC 1439417. For ESI and crystallographic data in CIF or other electronic format see DOI: 10.1039/c5sc04673d |
|
This journal is © The Royal Society of Chemistry 2016 |
Click here to see how this site uses Cookies. View our privacy policy here.