DOI:
10.1039/C6RA04817J
(Paper)
RSC Adv., 2016,
6, 61473-61481
Ammonia-oxidizing bacterial communities and shaping factors with different Phanerochaete chrysosporium inoculation regimes during agricultural waste composting
Received
23rd February 2016
, Accepted 19th June 2016
First published on 22nd June 2016
Abstract
This research was conducted to determine the effects of Phanerochaete chrysosporium inoculation on the ammonia-oxidizing bacterial (AOB) communities during agricultural waste composting. AOB communities with different inoculation regimes were investigated by quantitative PCR and denaturing gradient gel electrophoresis. Results showed that P. chrysosporium inoculation imposed certain stimulatory effects on the AOB amoA gene abundance. Samples with different inoculation regimes were dominated by different AOB species. Linear regression analysis indicated that the AOB community abundance had a significant positive correlation with pile pH (P < 0.05). The AOB amoA gene structure was best related to water soluble carbon (WSC) (P = 0.002, F = 14.17) and pile temperature (P = 0.04, F = 2.72). Variance partition analysis suggested that the sample property heterogeneity induced by inoculation imposed a greater impact (42.9%, P = 0.006) on the bacterial amoA gene structure than different inoculation regimes (23.6%, P = 0.022).
1. Introduction
Composting has become a major way to stabilize and process agricultural and municipal solid waste.1–3 Agricultural solid waste processed by composting can be utilized for other agricultural purposes to give economic, social and environmental benefits.4–6 Microbial communities play crucial roles during the composting process. However, agricultural waste contains a large amount of lignocelluloses and other aromatic compounds that are resistant to microbial degradation, which hampers the composting speed.7–9 Therefore, shortening the composting process and improving compost quality have been the focus of research. So far, many studies have been performed on the efficient degradation of lignin. Over 50 species of lignocellulolytic microorganisms have been identified from composting systems. Previously studied white-rot fungi included Phanerochaete chrysosporium, Trametes versicolor, Penicillium expansum, Pleurotus pulmonarius, Coriolus versicolor and others.7–10 Many studies have shown that the efficiency of composting could be improved by introducing lignocellulolytic microorganisms into the composting systems,8,9 ultimately improving the quality of the compost.10 White-rot fungi are regarded as major degraders of woody materials in terrestrial environments because of their excellent ability to decompose lignin.9
It has been reported that introducing exogenous white-rot fungi into the composting process can significantly alter nitrogen transfer and transformation.10–12 After inoculation with P. chrysosporium, accumulation of nitrate (NO3−-N) occurred at the late stage of agricultural waste composting,11,12 indicating that there were a large number of ammonia-oxidizing microbial communities, especially nitrite oxidizing bacteria in the agricultural waste composts. The symbiotic relationships between exogenous white-rot fungi and indigenous bacterial communities during the decomposition of lignocellulosic materials have attracted considerable attention around the world.11,13–16 Our previous study found that the inoculation with P. chrysosporium both during the first and second fermentation phase had stimulatory effects on the number of bacteria in the composting system.11 P. chrysosporium also affected the composition of bacterial communities mainly by improving pile temperature and the utilization of nutrients.11 However, little is known about how the white-rot fungi inoculation affects on the ammonia-oxidizing bacteria (AOB). Identifying and tracing the characteristics of the AOB community in the composting processes can help us to comprehensively understand the microbiological mechanisms of nitrogen balance in composting systems. AOB convert ammonia into nitrite, which is a necessary and rate-limiting reaction step for nitrification. AOB not only have a direct relationship with nitrogen transfer and transformation in composting systems but are also closely related to changes of ecological factors. It is of great importance to analyze the relationship between the changing characteristics of AOB communities in composting systems and a variety of biotic/abiotic factors.
In this study, we adopted different P. chrysosporium inoculation regimes, utilized the quantitative PCR and denaturing gradient gel electrophoresis (DGGE) to investigate how different P. chrysosporium inoculation regimes affect the AOB communities during agricultural waste composting. Research on the regulatory mechanism of changes in the indigenous ammonia-oxidizing bacterial community can deepen our understanding of microbial processes during agricultural waste composting, and thus improve inoculation strategies and shorten the composting process, to improve the composts quality.
2. Materials and methods
2.1 Fungus preparation and composting materials
P. chrysosporium strain (BKM-F-1767) was purchased from the China Center for Type Culture Collection (Wuhan University, China). Stock cultures were stored on the YMPG culture medium (malt extract 10 g, yeast extract 2 g, glucose 10 g, peptone 2 g, asparagine 1 g, KH2PO4 2 g, MgSO4·7H2O 1 g, thiamine-HCl 1 mg, distilled water 1000 mL) at 4 °C before use. The fungus was transferred to 500 mL Erlenmeyer flasks each containing 200 mL of potato-dextrose medium, and incubated at 37 °C on a rotary shaker at 135 rpm for 7 days. A sterile glass bead (8 mm) was added to each flask to break the big mycelia pellets that may occur during incubation into small ones (<3 mm). The culture was then filtrated and washed thrice with 200 mL of 0.12 M HaH2PO4–Na2HPO4 buffer. After measuring moisture contents, those prepared mycelia were used as inoculants.
2.2 Composting piles set-up
The typical agricultural wastes were collected from the suburb of Changsha, Hunan, China. Air-dried rice straw was cut into 10–20 mm lengths, and used as organic materials which were difficult to be decomposed. Several kinds of vegetables were collected from the student canteen of Hunan University, air-dried, chopped into 10–20 mm pieces, and used as easy-degradable materials. Soil was collected from the Yuelu Mountain, Changsha, sieved through a 40-mesh screen (0.38 mm) to remove coarse plant debris, and added to offer some necessary nutrients and increase microbial population. The chemical characterization of those materials has been reported previously.11,12 Rice straw, vegetables, soil, and rice hulls were homogenized at a ratio of 11
:
3
:
8
:
2 (fresh weight) and packed loosely in open boxes. The initial carbon/nitrogen (C/N) ratio and organic matter content of each pile were about 30
:
1 and 60%, respectively.
All the following four treatments (runs) were conducted in triplicate. Run A was the control without P. chrysosporium inoculants. Runs B and C were inoculated with 1% of P. chrysosporium mycelium (fresh weight) during the first fermentation phase (day 2) and the second fermentation phase (day 20), respectively. Run D was inoculated with the same amount of P. chrysosporium mycelium both during the first and the second fermentation phases. After inoculation, those composting piles were turned fully to spread the microbial consortium. The experiment was conducted for 50 days. Based on the pile temperature fluctuations, piles were turned twice a week during the first 2 weeks and once a week afterwards to provide aeration.
2.3 Sample collection and genomic DNA extraction
The subsamples for sample property analysis were collected from different places of the composting piles on days 0, 7, 14, 32 and 50, respectively, to discern the dynamic development of microbial communities. Physico-chemical factors, such as pile temperature, pH, C/N ratio, moisture, nitrate, ammonium, water soluble carbon (WSC), were determined according to the methods described previously.11,12 Samples for total DNA extraction were pooled at each sampling event, mixed and stored immediately at −20 °C before use. After sampling, the moisture content was measured and subsequently adjusted, to about 55–60% during the first fermentation phase and about 45–50% during the second fermentation phase by adding sterile deionized water. Genomic DNA of composting samples was extracted by using a PowerSoil kit (MoBio Laboratories, Carlsbad, CA, USA) following the manufacturer's instructions. The DNA solutions (approximately 100 μL for each extraction) were pooled to reduce sample variability, and stored at −20 °C before use.
2.4 Quantitative PCR of bacterial amoA gene
Quantitative PCR was conducted to estimate the bacterial amoA gene abundance by using primers amoA-1f (5′-GGGGTTTCTACTGGTGGT-3) and amoA-2r (5′-CCCCTCKGSAAAGCCTTCTTC-3) [K = G or T; S = G or C]17 for different inoculation treatments. Quantitative PCR was performed in triplicate on the iCycler IQ5 Thermocycler (Bio-Rad, USA) in a total volume of 20 μL containing 10 μL of 2 × SYBR real-time PCR premixture (Bioteke, Beijing), 0.5 μL of each primer (10 μM), 2 ng of compost microbial DNA. The amoA gene amplification was conducted using cycling conditions as follows: 95 °C for 2 min, followed by 40 cycles of 15 s at 95 °C, 30 s at 55 °C, 30 s at 72 °C and 20 s at 83 °C. Data was retrieved at 83 °C. Melting curve analysis was performed to verify amplification specificity. A negative control without the template DNA was included in every quantitative PCR assay. The amoA gene fragment pre-amplified from the composts DNA was cloned into the plasmid (pGEM-T Easy Vector, Promega, USA) and used as the standard for quantitative PCR after quantification with the BioPhotometer Plus (Eppendorf, Germany). The standard curve of bacterial amoA genes was linear over six orders of magnitude from 1.0 × 103 to 1.0 × 108 copies of template. The presence of PCR inhibitors in DNA extracted from composting samples was examined by diluting the DNA extract and mixing with a known amount of standard DNA before quantitative PCR.18 None inhibition was detected in any of the cases.
2.5 PCR-DGGE
PCR amplification of the bacterial amoA genes for DGGE analysis were conducted by using primers amoA-1f and amoA-2r mentioned above, with the forward primer (amoA-1f) attached a GC clamp to prevent complete separation of the DNA strands during DGGE.4 The PCR mixture was prepared with 1 μL of DNA extract, 1 μL of BSA (20 mg mL−1), 25 μL of 2 × Power Taq PCR MasterMix (Bioteke, Beijing), 1 μL of each primer (10 μM), and adjusted to a final volume of 50 μL by sterile water. PCR amplification was run on a MyCycler thermal cycle (Bio-Rad, USA) with the cycling conditions as follows: 4 min for 95 °C; 35 cycles of 95 °C for 20 s, 55 °C for 30 s, and 72 °C for 30 s; followed by 72 °C for 10 min, and end at 4 °C.
DGGE analysis of bacterial amoA gene was conducted by using the Dcode™ Universal Mutation Detection System (Bio-Rad, USA). Purified PCR products (∼30 μL) containing approximately equal amount of gene amplicons were loaded onto the 0.75 mm-thick 8% (w/v) polyacrylamide gel with a denaturing gradient of 30–50%. The electrophoresis was performed at 60 °C and 90 V for 12 h. After staining with Du-red nucleic acid gel stain, the gel was scanned with the Gel Doc XR System (Bio-Rad, Hercules, CA, USA). The band profile was digitized after average background subtraction for the entire gel. Band position and relative intensity within lane were quantified prior to further statistical analyses with the QuantityOne software (version 4.5, Bio-Rad, Hercules, CA, USA) as previously described.4
Bacterial amoA gene bands were excised and re-amplified by using primers without GC clamp mentioned above. PCR products of the expected size were excised from the 2.0% of agarose gel and purified with a DNA Fragment Purification Kit (Toyobo, Osaka, Japan). The gene fragments were ligated into the plasmid vector pGEM-T (Promega, USA) and transformed into Escherichia coli cell (DH5α). Blue-white colony appearance and ampicillin resistance were used to identify the positive clones. In total, 12 clone libraries were constructed. The plasmid DNA was extracted from each transformant by using the TIANpure Midi Plasmid Kit (Tiangen, Beijing, China) before sequencing (Majorbio Bio-Pharm Technology, Shanghai). Sequences with high identity were retrieved from NCBI for phylogenetic analysis. A neighbor-joining tree was constructed by using Kimura 2-parameter model as implemented in MEGA 5 program.19 Branch support was assessed by using 1000 bootstrap replicates.
2.6 Data analysis
Least-significant difference (LSD) test was performed to compare the mean values of physico-chemical factors and bacterial amoA gene abundance in different treatments. Linear regressions were determined to explore the potential distribution patterns of bacterial amoA gene abundance along gradients of sample properties. All of the above mentioned analysis were conducted by using the software package SPSS for windows (version 11.5, SPSS, Chicago, IL).
The correlation between the distributions of bacterial amoA gene structure and environmental factors during composting was assayed by redundancy analysis using Canoco version 4.5 (Centre for Biometry, Wageningen, The Netherlands).4,20 Forward selections were carried out to test which factor(s) had significant influence on the bacterial amoA gene structure. The selection procedures were stopped when the factor to be added was not significant anymore. Variation partitioning analysis was also performed to discriminate the influence of each significant factor.11 Monte Carlo reduced model tests with 499 unrestricted permutations were used to statistically evaluate the significance of the first canonical axis and of all canonical axes together. Statistical significance was defined at P < 0.05.
3. Results and discussion
3.1 Physico-chemical factors
The changes of different physico-chemical factors induced by different P. chrysosporium inoculation regimes during agricultural waste composting are shown in Table 1. Pile temperature for all treatments reached the peak values on day 7, with the values in Runs B and D inoculated during the first fermentation phase significantly higher than that in Runs A and C, indicating the biological activity was improved when the inoculants were present.9,10 The pH increased significantly during the first fermentation phase. Nitrate decreased to a low level on day 7, which might due to the high pile temperature and excessive amount of ammonia inhibited the activity and growth of nitrifying organisms.4,11 Our results are consistent with previous study,10 the pH and nitrate of Runs C and D were significantly higher than the no-inoculated treatments during the second fermentation phase. Moreover, P. chrysosporium inoculation significantly reduced the C/N ratio, WSC and ammonium during the entire composting process. C/N ratio of piles inoculated during the second fermentation phase (Runs C and D) decreased to 17.7 and 16.1 on day 32, while similar value were obtained in Run A but on day 50. The reason might be the quick mineralization of carbohydrates by P. chrysosporium that is characterized by its unique ability to degrade lignocellulose in composts.9 Overall, C/N ratio, ammonium and ammonium/nitrate ratio dropped to 13.6–16.9, 351.2–471.9 mg kg−1 (dry weight) and 0.199–0.340 on day 50, respectively, indicating the composting product was mature in this experiment.21 The composting process was accelerated by exogenous white-rot fungi especially during the second fermentation phase.
Table 1 Changes in physico-chemical factors for different inoculation treatments during agricultural waste compostinga
Treatments |
Days |
Pile temperature (°C) |
pH |
C/N |
Ammonium (mg kg−1) |
Nitrate (mg kg−1) |
WSC (mg kg−1) |
Moisture (%) |
Statistically significant differences of factors between samples at each sampling event are presented by lower-case letters (a, b, c, d) according to Least-significant difference (LSD) test (P < 0.05). C/N, total organic carbon/nitrogen ratio; WSC, water soluble carbon. |
Run A |
1 |
34.5a |
8.38a |
29.7a |
741.2a |
1062.8a |
266.8a |
64.7a |
7 |
51.5a |
8.14a |
26.6a |
1405.5a |
733.8a |
154.2a |
63.9a |
14 |
40.5a |
8.46a |
22.9a |
882.7a |
1155.4a |
107.7a |
66.8a |
32 |
31.0a |
8.76ab |
19.1a |
569.9a |
1219.6a |
80.2a |
54.5ab |
50 |
22.0a |
8.32a |
16.9a |
471.9a |
1382.9a |
68.2a |
56.0a |
Run B |
1 |
35.0a |
8.37a |
29.7a |
744.5a |
984.3a |
257.4a |
60.5a |
7 |
55.0b |
8.46b |
25.2a |
1554.6b |
701.6a |
140.6a |
56.7b |
14 |
43.5a |
8.62a |
21.0b |
933.2b |
1163.8a |
85.2b |
64.8a |
32 |
32.5a |
8.92bc |
17.7b |
474.3b |
1323.3b |
60.7b |
61.8c |
50 |
23.0a |
8.56b |
15.6b |
412.2b |
1444.8a |
52.8b |
53.6a |
Run C |
1 |
35.0a |
8.29a |
29.9a |
736.5a |
1038.7a |
259.6a |
61.5a |
7 |
52.5a |
8.33c |
25.8a |
1439.8a |
778.7b |
157.6a |
66.1a |
14 |
41.5a |
8.52a |
21.7a |
862.6a |
1112.6a |
103.6a |
65.2a |
32 |
34.0a |
8.99c |
17.7b |
492.3b |
1498.8c |
78.0a |
58.4bd |
50 |
24.0a |
8.85c |
15.2b |
377.7b |
1582.6b |
55.1b |
51.2b |
Run D |
1 |
36.0a |
8.32a |
30.1a |
728.4a |
1003.8a |
269.1a |
60.5a |
7 |
54.5b |
8.67b |
26.8a |
1621.2b |
694.4a |
144.7a |
56.8b |
14 |
43.0a |
8.78b |
20.6b |
999.6c |
1226.2a |
90.1b |
64.9a |
32 |
33.0a |
9.08c |
16.1c |
426.8c |
1666.0d |
65.6b |
60.3cd |
50 |
23.0a |
8.74c |
13.6c |
351.2b |
1756.7c |
42.4c |
57.2a |
3.2 AOB amoA gene abundance
P. chrysosporium inoculation at different phases imposed certain stimulatory effects on AOB amoA gene abundance (Fig. 1). The AOB amoA gene abundance on day 7 increased by 7–9 fold compared to the first day. During the first fermentation phase, amoA gene abundance was significantly increased in Runs B and D after inoculation with P. chrysosporium (P < 0.05, respectively) compared to the control treatment (day 14, Run A). AOB amoA gene abundance responded to P. chrysosporium inoculation during the second fermentation phase, with gene abundance in Run C and Run D higher than that in Run A. Furthermore, during the second fermentation phase, the bacterial amoA gene abundance in Run B, which was inoculated only at the first fermentation phase, showed no significant difference with that in Run A.
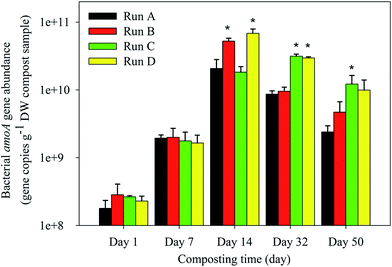 |
| Fig. 1 Changes in ammonia-oxidizing bacteria (AOB) amoA gene abundance under different inoculation treatments. The asterisk (*) above bars indicates significant differences between respective inoculation treatments and control (Run A) for different sampling times at P < 0.05 according to the least-significant difference method. | |
As for AOB, AOA also play important roles in nitrogen transfer and transformation in composting systems. It has been reported that a large number of AOA exist in cattle dung compost and they contain more amoA genes than AOB.6 Enriching chicken manure compost with the addition of AOA can increase pile temperature at the thermophilic stage, shorten the cycle of compost maturity and improve the nitrogen content of compost products.22 However, other studies have indicated that the archaeal amoA gene could not be detected at different stages of composting.5,23 In this study, AOA were not detected, indicating that the microbial communities were similar to those in the raw compost materials. It has been reported that high concentration of ammonium nitrogen are able to hamper the thermophilic nitrifying archaea from assimilating CO2, indicating that high-concentration ammonium nitrogen can inhibit ammonia oxidation.24 This may explain why AOA could not be detected in all stages of composting, while abundant AOB could be detected during the mesophilic stage in some cattle manure composts with a high-concentration of ammonium nitrogen.25
P. chrysosporium inoculation can influence nitrogen transfer and transformation in the composting process of agricultural waste. Several factors can affect the dynamics of AOB communities, subsequently drive nitrogen transfer and transformation in compost substrates. This may be ascribed to the fact that P. chrysosporium inoculation can increase microbial community abundance and improve their activities, thus induce the production of water soluble ammonia, creating a favorable environment for the survival of AOB and promoting their growth and reproduction. Our previous study found that the total bacterial 16S rDNA gene abundance increased significantly in all the four treatment groups up to 28–44 fold on day 7.11 Although P. chrysosporium inoculation induced an increase in AOB amoA gene abundance during the first fermentation phase, the AOB amoA gene abundance did not rise proportionally with an increase in the total bacterial community. This may be explained by the excessively high pile temperature (day 7, >50 °C) during the thermophilic stage inhibited the activity of AOB. Pile temperature is a critical factor influencing mutual adaptation between microbial communities and composting systems.4,11 During the second fermentation phase, the bacterial amoA gene abundance decreased slightly. However, the AOB abundance in Runs A and B was significantly lower than that of Runs C and D inoculated during the second fermentation phase (Day 32 and Day 50). The exogenous white-rot fungal species and the sample property heterogeneity induced by the different inoculation regimes provided an unfavourable condition for the AOB populations at this time. White-rot fungi colonize lignocellulose substrates and might compete directly with indigenous bacterial species for space and resources.11 Pile temperature and pH in Runs A and B without inoculation during the second fermentation phase were lower than that of Runs C and D. Ammonia in the compost substrate was converted to ammonium with decreasing pH, which directly influenced the ability of AOB to acquire ammonia substrates.
The stimulatory effect of P. chrysosporium inoculation on AOB amoA gene abundance was higher during the second fermentation phase. AOB amoA gene abundance in Run C and Run D were 3.3–6.1 fold higher than the control treatment (Run A), and this was probably because the excessively high pile temperature during the first fermentation phase was avoided. These conditions are favorable for growth and reproduction of ammonia-oxidizing microbes, thus, having a significant positive impact on AOB amoA gene abundance. In addition, several studies have shown that inoculation time is a crucial factor influencing the production and activity of lignocellulolytic enzymes during the composting process.9,11 Low-molecular-weight organic compounds were released into compost substrates through a variety of extracellular enzymes by P. chrysosporium. These compounds subsequently stimulated the growth and metabolism of the bacterial community.10,26 As a nutrient source that is easily utilized by microbes, WSC molecules might affect the growth and metabolic activity of different microbial community. Fermentative metabolism of microbes usually occurs in an environment with high WSC content, while respiratory metabolism occurs in an environment with trace concentration of WSC.27
3.3 Community structure of AOB
During the composting processes, the changes in AOB amoA gene structure under different P. chrysosporium inoculation regimes are shown in Fig. 2. Overall, different inoculation regimes resulted in differences in AOB community structure. There were different dominant AOB species at different stages of composting (Fig. 2). AOB amoA gene structure was similar in samples with different inoculation regimes at the thermophilic stage (day 7) and only several weak bands were detected. However, P. chrysosporium inoculation during the first fermentation phase significantly promoted the rapid reproduction of AOB community and improved the diversity of gene composition (Runs B and D, day 14). A total of 12 dominant amoA gene bands were purified, cloned and sequenced. Those dominant sequences all clustered to different species of the genus Nitrosomonas (Fig. 3). Inoculation during the first fermentation phase imposed an obvious stimulatory effect on species, with sequences of band 1, band 2 and band 4 belonging to Nitrosomonas europaea and an unknown Nitrosomonas cluster. Band 3 and band 6 had extremely similar sequences to Nitrosomonas nitrosa, which belongs to the Nitrosomonas communis cluster, was higher in Run A than in the inoculated treatments (Runs B, C and D). The results mentioned above indicated that P. chrysosporium inoculation at different times imposed a significant selective effect on the bacterial amoA gene structure during agricultural waste composting.
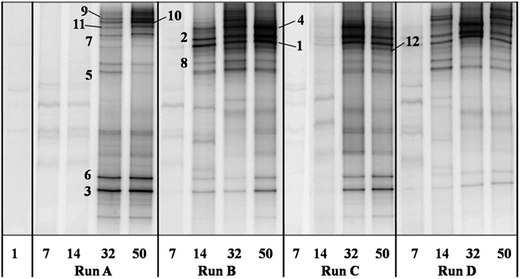 |
| Fig. 2 DGGE profiles of ammonia-oxidizing bacterial (AOB) amoA gene under different inoculation treatments. | |
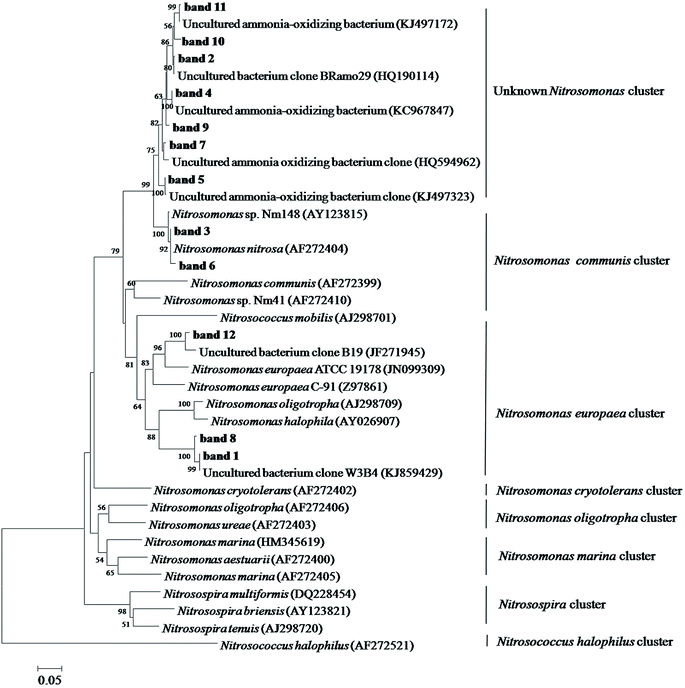 |
| Fig. 3 Phylogenetic tree of bacterial amoA gene sequences. Bootstrap values (>50%) are indicated at branch points. The scale bar represents 5% sequence divergence. Sequences from this study are depicted by bold letters. | |
AOB mainly belong to five genera including the Nitrosomonas, Nitrosovibrio, Nitrosolobus, Nitrosospira and Nitrosococcus.23 Many studies indicated that Nitrosospira and Nitrosomonas were the dominant species of AOB in composting systems.5,28,29 Nitrosomonas europaea/eutropha was the most dominant AOB species in the thermophilic stage during food waste composting.3 Our previous study showed that the bacterial amoA gene abundance decreased to undetectable level during the thermophilic and cooling stages during agricultural waste composting.30 They were also related to the microbial ammonia oxidation activity especially during the mesophilic and maturation stages.30 AOB community that mostly clustered with Nitrosomonas actively engaged in the biological oxidation of ammonia in composting processes.31 These results indicated that AOB played a crucial role during the waste composting process. As chemoautotrophic microbes, AOB have some limitations to their physiological characteristics, such as sensitivity to environmental factors and other exogenous microbial species inoculated in composts. Introducing exogenous P. chrysosporium might induce competition with indigenous AOB communities during composting through growth and reproduction or other effects, thus impacting the AOB community structure. Moreover, numerous studies indicated that sample properties of composts (e.g., pile temperature, C/N ratio, WSC) were significantly changed by white-rot fungi inoculation.8,10,11 Changes in the bacterial amoA gene structure due to different inoculation regimes might be closely related to the heterogeneity of the composting materials. Exogenous white-rot fungi inoculation can indirectly cause changes in the AOB community structure through changing physico-chemical factors such as temperature, pH, and different carbon and nitrogen sources at different stages. Confirming which factors significantly influence the AOB communities under different inoculation regimes is an important step to better interpret the changes occurring during waste composting system.
3.4 Factors influencing AOB communities under different inoculation regimes
Linear regression analysis showed that the AOB amoA gene abundance had a significant positive correlation with pile pH (P < 0.05) (Table 2). Redundancy analysis indicated that the temporal variation of the AOB gene structure was best related to WSC (P = 0.002, F = 14.17) and pile temperature (P = 0.04, F = 2.72) (Fig. 4). These factors as well as the interactions among them might have great impacts on the AOB community structure in samples with different P. chrysosporium inoculation regimes. Variance partition analysis, based on the redundancy analysis, suggested that changes in compost substrate conditions induced by different inoculation regimes imposed a greater impact (42.9%, P = 0.006) on the bacterial amoA gene structure than different inoculation regimes (23.6%, P = 0.022) (Fig. 5). The results mentioned above imply that P. chrysosporium inoculation influenced AOB amoA gene abundance and structure mainly by improving pile temperature, utilizability of nutrients in compost substrates and by changing other parameters.
Table 2 Pearson correlations between physico-chemical factors and ammonia-oxidizing bacterial amoA gene abundance during agricultural waste composting (*P < 0.05)a
|
Pile temperature |
pH |
C/N |
WSC |
Moisture content |
Ammonium |
Nitrate |
C/N, total organic carbon/nitrogen ratio; WSC, water soluble carbon. |
Pearson correlation coefficient |
0.067 |
0.511* |
−0.361 |
−0.428 |
0.313 |
−0.087 |
0.324 |
R2 |
0.004 |
0.261 |
0.130 |
0.183 |
0.098 |
0.008 |
0.105 |
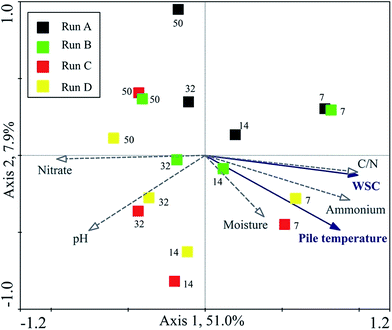 |
| Fig. 4 Redundancy analysis for bacterial amoA gene DGGE profiles. Significant composting factors are indicated by solid blue lines with filled arrows while not significant factors are shown using gray dotted lines with unfilled arrows. Numbers refer to the sampling days. Run A was the control without P. chrysosporium inoculants. Runs B and C were inoculated during the first and the second fermentation phase, respectively. Run D was inoculated with the same amount of P. chrysosporium mycelium both during the first and the second fermentation phase. WSC, water soluble carbon. | |
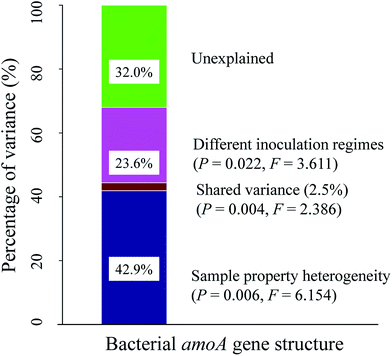 |
| Fig. 5 Variance decomposition of the variation partition analysis of DGGE profiles for bacterial amoA gene under different inoculation treatments. F and P values were estimated using Monte Carlo permutations. | |
The viable temperature and optimum temperature for the growth of P. chrysosporium was 20–50 °C and 36–40 °C, respectively.32,33 The higher pile temperature during the thermophilic stage (>50 °C) provided unfavourable conditions for P. chrysosporium population.12 Therefore, inoculation during the second fermentation phase (day 20) will help to avoid the extreme high-temperature for exogenous white-rot fungi, and ultimately promote the microbial activity in composts. The biological activity of the composting samples was improved when the inoculants were present.10–12 Previous research showed that the white-rot fungi inoculation changed the sample properties during agricultural waste composting.8,10,11 The pile temperature of Runs B and D during the first fermentation phase was significantly higher than that in Runs A and C which was not inoculated with P. chrysosporium. Pile temperature was regarded as an important factor affecting the dynamics of AOB communities. AOB had relatively high growth rate at 37 °C and 4 or 10 mM of ammonium in liquid cultures seeded with fermenting cattle manure composts, and the dominant AOB community of Nitrosomonas halophiles.34
Different AOB species might adapt to different nutrient conditions.4 Exogenous inoculants can indirectly influence the AOB community by affecting other factors such as C/N, pH, ammonium, etc.35 C/N and ammonium were significantly decreased in treatments that inoculated during the second fermentation phase. Higher bacterial/fungal community ratio was usually obtained in samples with lower C/N in compost.36 Without nitrogen limitation, the thermophilic stage with higher pile temperature maintained over a relative long time in samples inoculated with P. chrysosporium inoculation, which might favor thermophilic bacterial growth.11,12 Moreover, previous research revealed that the dynamics of the AOB community was mainly attributed to temporal changes in nitrate and the pH of the compost material (P < 0.05) during food waste composting.3 When pH decreased, ammonia in the compost substrate was converted to ammonium, which directly influenced the ability of AOB to acquire ammonia substrates. As the substrate is indirectly utilized, ammonium ions can significantly influence the community structure of AOB.37 Ammonium is widely considered to be linked to the abundance and categories of ammonia-oxidizing microbial communities. A higher content of ammonium generally inhibited the AOB growth possibly because free ammonia is toxic for most microbial cells in environments. A previous research showed that Nitrosomonas eutropha and Nitrosomonas eutropha-like species were tolerant to high ammonium content (ranging from 600 mM to 800 mM).38 High ammonium-tolerant AOB species have been widely cultivated from activated sludge and cattle manure compost.39,40
4. Conclusions
P. chrysosporium inoculation imposed stimulatory effects on the AOB amoA gene abundance during agricultural waste composting. Samples with different inoculation regimes were dominated by different AOB species. AOB amoA gene abundance had a significant positive correlation with pH (P < 0.05). The AOB gene structure was best related to WSC (P = 0.002) and pile temperature (P = 0.04). Variance partition analysis indicated that the sample property heterogeneity induced by inoculation imposed a greater impact (42.9%, P = 0.006) on the bacterial amoA gene structure than different inoculation regimes (23.6%, P = 0.022).
Acknowledgements
This work was supported by the National Natural Science Foundation of China (51408219, 51108423, 51108178, 51509233), and Special Fund of Scientific Research of The Education Department of Hunan Province (14C0565) and Science Foundation for Young Scientists of Hunan Agricultural University (14QN22).
References
- C. Wu, Y. Li, W. Li and K. Wang, RSC Adv., 2015, 5, 95960–95966 RSC.
- G. Zeng, H. Wu, J. Liang, S. Guo, L. Huang, P. Xu, Y. Liu, Y. Yuan, X. He and Y. He, RSC Adv., 2015, 5, 95960–95966 RSC.
- S. Shi, D. Zou, Q. Wang, X. Xia, T. Zheng, C. Wu and M. Gao, RSC Adv., 2016, 6, 9541–9548 RSC.
- J. Zhang, G. Zeng, Y. Chen, M. Yu, Z. Yu, H. Li, Y. Yu and H. Huang, Bioresour. Technol., 2011, 102, 2950–2956 CrossRef CAS PubMed.
- K. Maeda, S. Toyoda, R. Shimojima, T. Osada, D. Hanajima, R. Morioka and N. Yoshida, Appl. Environ. Microbiol., 2010, 76, 1555–1562 CrossRef CAS PubMed.
- N. Yamamoto, K. Otawa and Y. Nakai, Microb. Ecol., 2010, 60, 807–815 CrossRef CAS PubMed.
- D. L. Huang, G. M. Zeng, C. L. Feng, S. Hu, M. H. Zhao, C. Lai, Y. Zhang, X. Y. Jiang and H. L. Liu, Chemosphere, 2010, 81, 1091–1097 CrossRef CAS PubMed.
- M. Yu, G. Zeng, Y. Chen, H. Yu, D. Huang and L. Tang, Process Biochem., 2009, 44, 17–22 CrossRef CAS.
- G. Zeng, M. Yu, Y. Chen, D. Huang, J. Zhang, H. Huang, R. Jiang and Z. Yu, Bioresour. Technol., 2010, 101, 222–227 CrossRef CAS PubMed.
- H. Wang, B. Fan, Q. Hu and Z. Yin, Bioresour. Technol., 2011, 102, 11189–11193 CrossRef CAS PubMed.
- J. Zhang, G. Zeng, Y. Chen, M. Yu, H. Huang, C. Fan, Y. Zhu, H. Li, Z. Liu, M. Chen and M. Jiang, Appl. Microbiol. Biotechnol., 2013, 97, 3159–3169 CrossRef CAS PubMed.
- J. Zhang, G. Zeng, Y. Chen, J. Liang, C. Zhang, B. Huang, W. Sun, M. Chen, M. Yu, H. Huang and Y. Zhu, Biodegradation, 2014, 25, 669–680 CrossRef CAS PubMed.
- V. Hervé, E. Ketter, J. C. Pierrat, E. Gelhaye and P. Frey-Klett, PloS ONE, 2016, 11, e0147100 CrossRef PubMed.
- O. Skyba, D. Cullen, C. J. Douglas and S. D. Mansfield, Appl. Environ. Microbiol., 2016, 82 DOI:10.1128/AEM.00134-16.
- V. S. Varma, K. Ramu and A. S. Kalamdhad, Environ. Sci. Pollut. Res., 2015, 22, 7851–7858 CrossRef CAS PubMed.
- C. Zhou, Z. Liu, Z. L. Huang, M. Dong, X. L. Yu and P. Ning, Waste Management, 2015, 40, 38–43 CrossRef CAS PubMed.
- J. H. Rotthauwe, K. P. Witzel and W. Liesack, Appl. Environ. Microbiol., 1997, 63, 4704–4712 CAS.
- M. Kramer, N. Obermajer, B. B. Matijašić, I. Rogelj and V. Kmetec, Appl. Microbiol. Biotechnol., 2009, 84, 1137–1147 CrossRef CAS PubMed.
- K. Tamura, D. Peterson, N. Perterson, G. Stecher, M. Nei and S. Kumar, Mol. Biol. Evol., 2011, 28, 2731–2739 CrossRef CAS PubMed.
- J. Lepš and P. Šmilauer, Multivariate Analysis of Ecological Data Using CANOCO, Cambridge University Press, United Kingdom, Cambridge, 2003, pp. 43–75 Search PubMed.
- M. P. Bernal, J. A. Alburquerque and R. Moral, Bioresour. Technol., 2009, 100, 5444–5453 CrossRef CAS PubMed.
- K. Xie, X. Jia, P. Xu, X. Huang, W. Gu, F. Zhang, S. Yang and S. Tang, Bioresour. Technol., 2012, 120, 70–77 CrossRef CAS PubMed.
- U. Purkhold, A. Pommerening-Roser, S. Juretschko, M. C. Schmid, H. P. Koops and M. Wagner, Appl. Environ. Microbiol., 2000, 66, 5368–5382 CrossRef CAS PubMed.
- R. Hatzenpichler, E. V. Lebedeva, E. Spieck, K. Stoecker, A. Richter, H. Daims and M. Wagner, Proc. Natl. Acad. Sci. U. S. A., 2008, 105, 2134–2139 CrossRef CAS PubMed.
- T. Yamada, S. Araki, W. Ikeda-Ohtsubo, K. Okamura, A. Hiraishi, H. Ueda, Y. Ueda, K. Miyauchi and G. Endo, Syst. Appl. Microbiol., 2013, 36, 359–367 CrossRef CAS PubMed.
- P. Castaldi, G. Garau and P. Melis, Waste Management, 2008, 28, 371–378 CrossRef PubMed.
- K. Ishii and S. Takii, J. Appl. Microbiol., 2003, 95, 109–119 CrossRef CAS PubMed.
- Å. Jarvis, C. Sundberg, S. Milenkovski, M. Pell, S. Smårs, P. E. Lindgren and S. Hallin, J. Appl. Microbiol., 2009, 106, 1502–1511 CrossRef PubMed.
- G. A. Kowalchuk, Z. S. Naoumenko, P. J. L. Derikx, A. Felske, J. R. Stephen and I. A. Stephen, Appl. Environ. Microbiol., 1999, 65, 396–403 CAS.
- G. Zeng, J. Zhang, Y. Chen, Z. Yu, M. Yu, H. Li, Z. Liu, M. Chen, L. Lu and C. Hu, Bioresour. Technol., 2011, 102, 9026–9032 CrossRef CAS PubMed.
- N. Yamamoto, R. Oishi, Y. Suyama, C. Tada and Y. Nakai, Microbes Environ., 2012, 4, 519–524 CrossRef.
- J. Mouchacca, Cryptogam.: Mycol., 1997, 18, 19–69 Search PubMed.
- M. Tuomela, M. Vikman, A. Hatakka and M. Itavaara, Bioresour. Technol., 2000, 72, 169–183 CrossRef CAS.
- R. Oishi, C. Tada, R. Asano, N. Yamamoto, Y. Suyama and Y. Nakai, Environ. Microbiol., 2012, 63, 787–793 CAS.
- S. Avrahami, L. Werner and C. Ralf, Environ. Microbiol., 2003, 5, 691–705 CrossRef CAS PubMed.
- F. Eiland, M. Klamer, A. M. Lind, M. Leth and E. Baath, Microb. Ecol., 2001, 41, 272–280 CrossRef CAS PubMed.
- Y. Okano, K. R. Hristova, C. M. Leutenegger, L. E. Jackson, R. F. Denison, B. Gebreyesus, D. Lebauer and K. M. Scow, Appl. Environ. Microbiol., 2004, 70, 1008–1016 CrossRef CAS PubMed.
- H. P. Koops, B. Bottcher, U. C. Moller, A. Pommerening-Roser and G. Stehr, J. Gen. Microbiol., 1991, 137, 1689–1699 CrossRef CAS.
- A. Princic, I. Mahne, F. Megusar, E. A. Paul and J. M. Tiedje, Appl. Environ. Microbiol., 1998, 64, 3584–3590 CAS.
- T. Nakagawa and R. Takahashi, Microbes Environ., 2015, 30, 221–227 CrossRef PubMed.
|
This journal is © The Royal Society of Chemistry 2016 |