Self-sustained high-rate anammox: from biological to bioelectrochemical processes
Received
16th June 2016
, Accepted 11th September 2016
First published on 13th September 2016
Abstract
The slow growth rate of anammox bacteria is a pressing problem for system efficiency and stability. An innovative solution was explored in this study that involves accelerating anammox in microbial electrolysis cells (MECs) and alleviating their dependence on anammox bacteria. Batch tests showed that 85% of total nitrogen (TN) was removed in the MEC system, whereas only 62% of TN was removed in conventional anammox. Simulation of the modified Nernst–Monod model revealed that the maximum specific utilization rate (0.30 to 0.38 mmol g−1 VSS h−1) in the anammox MEC was 60% higher than in conventional anammox (0.18 to 0.20 mmol g−1 VSS h−1). Harvesting the power generated in microbial fuel cells (MFCs) to support the MECs substantially saved energy consumption and effectively utilized the low power output of MFCs. Simulation of the power management system (PMS) interface demonstrated the charge/discharge cycles for power supply by the MFCs and for power consumption by the MECs. The integrated MEC-MFC system accelerated anammox, required no external carbon source, effectively utilized wastewater energy, and thus achieved self-sustained nitrogen removal.
Water impact
This pioneering study provides a practical solution to a persistent drawback of anammox: the slow growth of anammox bacteria. Unique integration of MECs and MFCs alleviates dependence on anammox bacteria and accelerates total nitrogen (TN) removal efficiency to a level 30% higher than that of conventional anammox. A novel bioelectrochemical anammox kinetic model is developed. This study greatly improves the scientific understanding and engineering applications of energy-positive high rate biological nutrient removal processes.
|
Introduction
Nitrogen in wastewater is normally removed using biological nitrogen removal (BNR) processes consisting of aerobic nitrification and anoxic denitrification. However, conventional BNR is energy-negative and carbon-intensive due to the requirements of high aeration during nitrification, pH adjustments, long sludge retention times (SRT), and exogenous organic carbon in denitrification.1 In the past decade, anaerobic ammonium oxidation (ANAMMOX) has been developed; in this process, ammonium (NH4+) and nitrite (NO2−) are directly reacted to form nitrogen gas (N2) by anoxic anammox bacteria, which possesses unique advantages over conventional BNRs, including the lack of requirement for aeration and external carbon sources.2 Nevertheless, anammox has some drawbacks, including the long growth time (doubling time of 11 days) of anammox bacteria and difficult cultivation; thus, it is rarely used in full-scale wastewater treatment plants.3
Bioelectrochemical systems (BES) have drawn global attention for converting the chemical energy stored in wastewater to electrical energy.4–10 Microbial fuel cells (MFCs) utilize the difference between anode and cathode potentials as the driving force for electricity generation in spontaneous reactions (e.g. oxygen reduction, metal (Cr6+, Cu2+) reduction) and have been studied as a means to reduce the energy cost of denitrification, with nitrite and nitrate as the electron acceptors in the cathode chamber.11–16 On the other hand, microbial electrolysis cells (MECs) where the difference between the anode and cathode potentials is deficient can be supported with an external power supply (voltage < 1.0 V) to cause non-spontaneous reactions to proceed and to accelerate spontaneous reactions. For instance, chromium(VI) reduction was accelerated in MECs by changing the cathode potential.17 Nitrogen removal with nitrification on the anode and denitrification on the cathode was achieved in MECs.18 A previous study found that ammonium served as the anodic fuel in MFCs, and current generation was promoted during the process of electron transport from ammonium to nitrite.19 Therefore, installing a power supply in anammox MECs could accelerate ammonium oxidation.
The limited electric power output (less than 5 W m−2) of MFCs has posed a problem for their use as sole power sources.20,21 Thus, efficient utilization of low MFC power is critical for their real-world applications. In the past several years, MFCs have been studied as power sources for low power subsea devices and water quality sensors, and MECs have been used for hydrogen production and metal treatment.13,22–25 In particular, the low voltage requirement of MECs (<0.5 V) means that MFCs can be used as their power supplies. However, the interface between power production in MFCs and power consumption in MECs has not been established; thus, it is difficult to predict the power supply and consumption between MFCs for MECs.
Reliable estimation of kinetic parameters in anammox modeling is critical to better understand the mechanisms of anammox and enhance its performance. Monod, pseudo first order model and Haldane-type model kinetics have been used for anammox simulations.26–29 A wide range of half-velocity constants (0.003 to 13.7 mM) and maximum specific utilization rates (0.09 to 3.74 mmol N g−1 VSS h−1) has been obtained.29 With two reactants (ammonium and nitrite) being involved in anammox reactions, the Monod model with multiple and/or dual substrates should be used, as it can reflect the dependence of each substrate in the reactions. Furthermore, the Nernst–Monod model has been developed for electron donation and acceptance between substrates and biofilm electrodes in MFCs and MECs; this model has also been modified for the reduction of nitrate and nitrite in the cathode.30,31 To date, no model exists for anammox in MECs or MFCs. Due to the complexity of redox reactions and multiple substrates involved in anammox, a novel Nernst–Monod model coupled with a multi-substrate Monod model should be developed to simulate anammox in BES systems and the dependence of each substrate.
The purpose of this study was to accelerate anammox in MECs without solely relying on anammox bacteria and to explore the feasibility of the integrated MFC-MEC in wastewater treatment plants. MECs were powered by MFCs to treat wastewater and achieve self-sustained nitrogen removal without extra energy input while efficiently utilizing the low power output of MFCs, which no existing BNR or BES has accomplished. There were four tasks in this study. First, ammonium and nitrite were fed to MECs to examine their degradation rates. The accelerated anammox mechanisms in the MECs were verified by conducting three control tests (conventional anammox, ammonium/nitrite alone, and abiotic MEC test). Second, a kinetic model of accelerated anammox in the MECs was developed to fundamentally understand self-sustained anammox and the dependencies of ammonium and nitrite in anammox reactions. Third, the variation of important parameters (e.g. pH, redox potential) was examined to determine the occurrence of anammox in MECs and relate it to the nitrogen removal rate. Finally, the interface of the integrated MFC-MEC system was modeled to predict the power supply of MFCs to anammox MECs. The energy saving of the integrated system was calculated and compared with traditional BNR and anammox processes to confirm the effectiveness of this novel self-sustained anammox MFC-MEC with high nitrogen removal rate and minimal energy consumption.
Materials and methods
The anammox MEC setup
The batch-mode anammox MEC consisted of an anode chamber (volume: 140 mL) and a cathode chamber (volume: 140 mL) separated by a proton exchange membrane (N117, DuPont Fuel Cells, DE) (Fig. 1: anammox MEC). During the acclimation period, the anammox MEC was powered by a programmable power supply (model 3645A; Circuit Specialists, Inc.) with a stable voltage output of 0.5 V. The power supply was later replaced during the experimental period by two single-chamber microbial fuel cells (SCMFCs) (each volume: 140 mL) connected in series. The anode of the anammox MEC was connected with the cathode of the SCMFCs, and the cathode of the anammox MEC was connected with the external resistance (Rext 5 Ω) in the circuit connecting the anode of the SCMFCs. The voltages across the Rext were continuously recorded every 120 s using a Keithley 2700 data logging system. Carbon brushes (4 cm long by 4 cm diameter as projected area, Mill-Rose Carbon Fiber Brush) were used as the anode and cathode of the anammox MEC and the anode of the SCMFCs. Carbon cloth (4 × 4 cm2, Fuel Cell Earth LLC, MA) loaded with platinum (Pt) (0.5 mg cm−2) was used as the cathode of the SCMFCs.
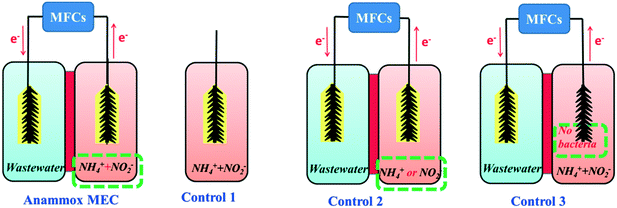 |
| Fig. 1 Diagrams of the integrated MFC-MES system and control setups. | |
Inoculation and operation of anammox MEC and control experiments
The anode chamber of the anammox MEC was inoculated with a mixture of aerobic and anoxic sludge (volume ratio: 1
:
1) from the University of Connecticut Wastewater Treatment Plant (UConn-WWTP). The anode was fed with ammonium chloride (NH4Cl, ∼50 mg-N L−1), sodium nitrite (NaNO2, ∼40 mg-N L−1), and the medium solution (0.2 mM Na2HPO4, 0.1 mM NaH2PO4, 0.2 mM KCl, 0.2 mM MgSO4·7H2O, and 6 mM NaHCO3) to stimulate the anammox bacteria growth. The inoculation lasted five weeks; half of the anodic solution (around 70 ml) was changed weekly and refilled with fresh ammonium, nitrite and nutrient solution. When the experiment started, the initial volatile suspended solids (VSS) concentration was about 0.4 g L−1 in the anammox MECs. The cathode was fed with the influent wastewater from UConn-WWTP. Both the anode and cathode were sealed with plastic caps to secure anaerobic conditions and prevent the occurrence of nitrification. The SCMFCs were filled with a mixture of the influent wastewater from UConn-WWTP and sodium acetate (15 mM) with chemical oxygen demand (1500 mg L−1) and operated for more than one month to provide stable voltage (0.5 V) for the anammox MECs.
Along with the anammox MEC experiment, three control experiments were performed side by side for comparison. Control 1 (conventional anammox) was conducted in a sealed plexiglass bottle (volume: 140 mL) with a carbon brush for biofilm growth (Fig. 1: Control 1). No power supply or SCMFC was connected. Control 2 had a similar setup to the anammox MEC, except it was fed with either ammonium or nitrite solution (Fig. 1: Control 2) to examine the nitrogen removal under single nitrogen species and the dependence of ammonium and nitrite for anammox and denitrification, respectively. Control 3 (abiotic test) was a similar setup to the anammox MFC, except that no sludge was inoculated in the anode (Fig. 1: Control 3) to compare with Control 1 (biotic anammox tests) and determine the role of bacteria in nitrogen removal in MECs. All the anammox MEC and control tests were carried out at 30 °C in duplicate.
Analysis of anammox MEC and control experiments
Ammonium concentration, nitrite, nitrate, chemical oxygen demand (COD), and alkalinity were measured with a spectrophotometer (DR 2800, HACH company, CO) and the TNTplus™ series (HACH company, CO). The pH in anammox MEC and controls were measured with a portable pH meter (Thermo Fisher Scientific Orion 3-star). The redox potential (ORP) of the anammox MEC and control experiments were measured with an ORP meter (Thermo Fisher Scientific Orion 3-star). The open circuit potentials (OCPs) of the anodes and cathodes were measured using Ag/AgCl (+197 mV vs. SHE) as the reference.
Modeling anammox MEC and conventional anammox
In order to predict the variation of ammonium and nitrite concentrations in the anammox MEC and estimate the kinetic parameters, the modified Nernst–Monod equation (eqn (1) and (2)) from previous studies was used,30,31 assuming that ammonium and nitrite (nitrate was negligible in this study) were the electron donors and that anode electrode was the electron acceptor. Meanwhile, Monod kinetics of double substrates was used to simulate nitrogen removal in conventional anammox without a power supply (Control 1) (eqn (3) and (4)). |  | (1) |
|  | (2) |
|  | (3) |
|  | (4) |
where [NH4+] and [NO2−] are the effluent concentrations of ammonium and nitrite (mg-N L−1); μm is the maximum specific utilization rate (mg N g−1 VSS h−1); Ks is the half-velocity constant (mg-N L−1); F is Faraday's constant (9.64853 × 104 C mol−1); R is the gas constant (8.314 J K−1 mol−1); T is the operation temperature (K); and η is the local potential (V), which was estimated as 0.2 V in this study.27
The results of the ammonium and nitrite concentrations in the anammox MEC and Control 1 over the experimental period were fit into the models (eqn (1) to (4)) to calculate critical kinetic parameters (maximum specific utilization rate and half-velocity constant). Integration of the differential equations was conducted using Matlab software (R2014a).
Modeling power support and consumption of the integrated MFC-MEC
The power support and consumption between the anammox MEC and the SCMFCs was simulated. The power output from the MFCs was evaluated based on total coulombs (Q), and the current produced during the operational period (t) was determined based on the organics consumption (ΔCOD) in wastewater (eqn (5)). Coulombs from SCMFCs (Q) were consumed in anammox MEC through the anammox pathways, so that the concentration of removed nitrogen (ΔCN) could be simulated with the number of electrons transferred per mole of nitrogen (NH4+) (eqn (6)). By establishing the interface of power generation in the SCMFCs and the power consumption in the anammox MEC, ΔCOD in the SCMFCs and ΔCN in the anammox MEC can be simulated. | 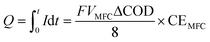 | (5) |
|  | (6) |
where Q is the total coulombs harvested from the MFCs (C); VMFC and VMEC are the SCMFC volume and MEC cathode volume (L); ΔCOD is the total COD consumed in the SCMFCs, (g L−1); CEMFC is the coulombic efficiency of the SCMFC (10 to 27%),32 with 20% being used in eqn (5); M is the molecular weight of nitrogen (14 g mol−1); n is the electron transfer per mole of nitrogen; and F is Faraday's constant (9.64853 × 104 C mol−1).
Results and discussion
Variation of ammonium and nitrite concentrations in MECs
Ammonium (NH4+) and nitrite (NO2−) concentrations gradually decreased in the MEC with a power supply of 0.5 V (Fig. 2). The initial concentrations of ammonium and nitrite were set at 50 mg L−1 and 40 mg L−1, respectively, to simulate domestic wastewater and the effluent after short-cut nitrification (ammonium to nitrite).16 10 days were required to remove 90% of the ammonium (average concentration below 5 mg L−1 in the effluent) and remove 85% of the nitrite (average concentration below 6 mg L−1 in the effluent). Nitrite decreased rapidly during the first 4 days; the decrease then slowed. Nitrate (NO3−) in the MEC was low (less than 5 mg L−1) and originated from the acclimated sludge residue taken from the anoxic tank of the UConn WWTP. The continuous nitrogen removal under anaerobic conditions (DO < 0.1 mg L−1, with both the anode and cathode being sealed) indicated the biotic process of this experiment, especially the function of anammox bacteria, because ammonium and nitrite could hardly be volatilized in the sealed system, nitrification could not occur under anaerobic conditions, and the anammox reaction could only take place with bacteria. The average nitrogen removal rate was 8.2 mg-N L−1 d−1, which was in the range of the reported values in BES (3–51 mg-N L−1 d−1), but was much lower than those in BNR (50 to 400 mg-N L−1 d−1).14,33–35 The main reason was the difference in substrate transfer in these systems. Conventional BNR is mainly operated in continuous flow mode; however, lab-scale BES (e.g. MEC, MFC) is normally operated in batch mode, with limited substrate diffusion from wastewater to the biomass.36
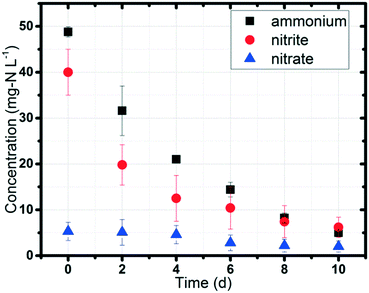 |
| Fig. 2 Variation of nitrogen concentration in the MEC anode over time. | |
Control tests to verify the occurrence of anammox and denitrification in MECs
Three control tests were conducted in order to further elucidate the removal pathways of ammonium and nitrite in MECs. All the control tests were operated for 10 days (the same duration as the MEC tests). Control test 1 (ammonia + nitrite, without power supply) simulated the anammox process (Fig. 1 Control 1); the average ammonium, nitrite and nitrate removal rates were 2.80 mg-N L−1 d−1, 3.24 mg-N L−1 d−1, and 0.44 mg-N L−1 d−1, respectively (Fig. 3). In contrast, the rates for the anammox MEC were 4.38 mg-N L−1 d−1, 3.38 mg-N L−1 d−1, and 0.33 mg-N L−1 d−1, respectively (Fig. 3). The reason for the lower ammonium removal rate in Control 1 than in MEC was that the power supply in the MEC expedited the ammonium removal, while ammonium removal in Control 1 was solely dependent on anaerobic ammonium oxidation by nitrite. This indicated that ammonium could function as the anodic fuel in MECs and transfer electrons to the anode in addition to nitrite. In this study, the high potential provided by the power supply resulted in more ammonium oxidation in the MECs than in Control 1, implying that the MECs had other electron acceptors for ammonium oxidation compared with the traditional anammox process. This finding was in accordance with a previous study, wherein the generation of electricity by ammonium oxidation possessed negative Gibbs free energy.19 In addition, ferric ion was found to function as an electron acceptor by anammox bacteria without nitrite,37 and nitrate-dependent ferrous iron was oxidized by anammox bacteria;38 this indicates that anammox bacteria can utilize different types of electron donors and acceptors and that anammox involves numerous complicated processes. In contrast with the ammonium removal difference between MEC and Control 1, the nitrite removal rates were similar between Control 1 and MEC (Fig. 3), implying that nitrite removal was not related to the power supply.
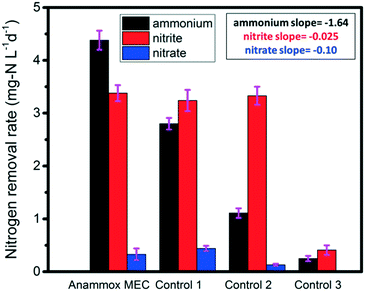 |
| Fig. 3 Nitrogen removal rates in the anammox MEC and control tests (inserted values are the slopes of the linear regression of average removal rates). | |
Control 2 was a test of single nitrogen species (ammonium or nitrite) with a power supply in order to elucidate whether ammonium and nitrite should be co-present in wastewater for the enhanced anammox-like process in MECs (Fig. 1 Control 2). The ammonium removal rate without nitrite input was 1.12 mg-N L−1 d−1 (Fig. 3), which was much lower than those of MEC and Control 1, while the nitrite removal rate without ammonia input was 3.28 mg-N L−1 d−1 (Fig. 3), which was similar to those of the MEC test and Control 1. Furthermore, the slopes of linear regression of the ammonium, nitrite and nitrate removal rates were analyzed for the MEC, Control 1, and Control 2, showing an obvious change in the ammonium removal rate compared with those of nitrite and nitrate (Fig. 3). The slope of ammonium data from the MEC to Control 2 was −1.64, indicating that the MEC was effective at ammonium removal, while the slope of nitrite was only −0.025, indicating that there was literally no change in nitrite removal among these three systems. These results implied the co-presence of nitrite facilitated ammonium oxidation, and nitrite was the main oxidant of ammonium. In contrast, nitrite removal was not affected by the absence of ammonium, indicating that nitrite could be reduced by anammox bacteria as well as by denitrifying bacteria. The denitrification process was dominant in the absence of ammonium. The Control 2 results implied that ammonium oxidation and nitrite reduction were carried out by anammox bacteria and/or anaerobic ammonium oxidation bacteria and denitrification bacteria. This synergic correlation accelerated the electron transfer from ammonium to nitrite and ultimately to the anode.
Control 3 (ammonium + nitrite with power supply, abiotic test) was conducted to verify the role of bacteria in anammox MECs (Fig. 1 Control 3). Both the ammonium and nitrite removal rates (0.25 mg-N L−1 d−1 and 0.41 mg-N L−1 d−1) were much lower than those of MEC, Control 1 and Control 2 (Fig. 3), indicating that the removal of ammonium and nitrite was a biological process and that bacteria carried out the ammonium oxidation and nitrite reduction. All the MEC and control tests had low nitrate concentrations (<5 mg L−1, mainly originating from raw wastewater) and low nitrate removal rates (less than 0.49 mg-N L−1 d−1) (Fig. 3), indicating that nitrification hardly occurred in anoxic conditions. Compared with complete nitrification (ammonium to nitrite and then to nitrate), short-cut nitrification (ammonium to nitrite) saved substantial reaction time, provided the essential electron acceptors (nitrite) in the subsequent anammox, and preserved carbon sources for partial denitrification (nitrite reduction).
The simultaneous removal of ammonium and nitrite under anoxic conditions in 10 days (Fig. 2) confirmed the occurrence of anammox in the MEC system. Nitrification was completely inhibited under anoxic conditions (DO < 0.1 mg L−1, with both the anode and cathode being sealed), which was verified by the stable decrease of nitrite (no accumulation) over the 10 day period. This provided clear evidence that ammonium oxidation was carried out by anammox bacteria with nitrite as the electron acceptor. Several studies of wastewater treatment by anammox MFCs had similar configurations (two chamber systems), inoculation protocols (wastewater and nutrient ingredients), and operational durations (5 to 14 days) to this study, along with the same decreasing trend in ammonium and nitrite;39–42 among these reports, some validated the anammox reactions through engineering tests,39,42 and some confirmed the existence of anammox bacteria by microbial community analysis (e.g. anammox 16S rRNA gene as a molecular marker and functional biomarkers (e.g. nitrite reductase gene) involved in the anammox metabolisms).40,41 Furthermore, simultaneous removal of ammonium and nitrite in anaerobic and anoxic units has been observed in traditional anammox systems (not BES) treating various types of wastewater (e.g. municipal wastewater, swine wastewater, landfill leachate, salinity wastewater) in bioreactors (e.g. sequencing batch reactor, rotating biological contactor, upflow anaerobic sludge blanket (UASB), moving bed biofilm reactor);43–51 among these, some studies confirmed the occurrence of anammox reactions through biochemical measurements and engineering operations,43–48 and some detected anammox bacteria using microbial analysis (e.g. cloning with anammox gene primers and gene sequencing, fluorescence in situ hybridization (FISH) with specific anammox bacterial probes).36,49–51 By comparing the experimental results in this work and those of previous anammox studies, the existence of anammox bacteria in the MFCs was ascertained.
Kinetic modeling of the accelerated anammox in MECs
The experimental data of the ammonium and nitrite concentrations in the anammox MEC and Control 1 (conventional anammox) were fitted with the models shown in eqn (1) to (4) to determine critical kinetic parameters. Specifically, the modified Nernst–Monod equation was used for anammox MEC (Fig. 4a), even though not all of the electrons were accepted by the electrode but were consumed by other processes (e.g. anammox and denitrification). The total nitrogen concentration change was measured over time (Fig. 4b). Multiplicative Monod modeling with dual substrates was used for Control 1 (Fig. 4c). The fittings of the model-simulated data and the experimental results were examined using statistical analysis. The coefficients of determination (R2) of the experimental results and the model-simulated data were all higher than 97% (ammonium in MECs: 99.12%, nitrite in MECs: 98.68%, ammonium in Control 1: 97.24%, and nitrite in Control 1: 99.48%). In addition, the models were examined by the difference (the subtraction of the predicted data from the observed or actual data) between the ammonium and nitrite experimental data in the MEC and Control 1 (the MEC experiment was duplicated 4 times, as shown in Fig. 4a; Control 1 was duplicated twice, as shown in Fig. 4c) to validate the model fitness (the inserted figures in Fig. 4). The distribution of the differences over the independent variable (time) indicated that they were randomly distributed and tended to cluster towards the middle of the plots (y = 0) in both the MEC and Control 1. In addition, the maximum difference was single digits (MEC maximum difference: 6.0 and −7.3; Control 1 maximum difference: 5.2 and −6.0) without high bias, demonstrating that the models fitted well to the experimental data.
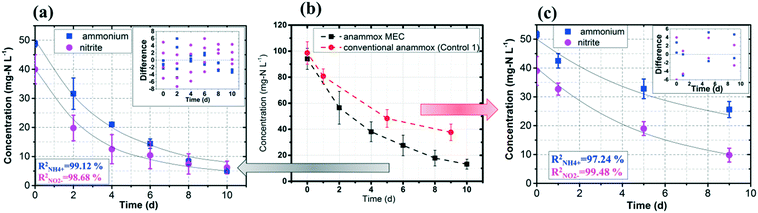 |
| Fig. 4 Variation of total nitrogen, ammonium and nitrite concentrations in anammox MEC and conventional anammox (Control 1) over time. a: Ammonium and nitrite concentrations in anammox MEC based on model simulations. b: Experimental results of total nitrogen concentration. c: Ammonium and nitrite concentrations in conventional anammox (Control 1) based on model simulations. The inserted figure is the difference between the observed data for ammonium and nitrite over time). | |
The calculated maximum specific utilization rate of ammonium in the MEC was much higher (0.38 mmol N g−1 VSS h−1) than that in Control 1 (0.18 mmol N g−1 VSS h−1) (Table 1). The maximum specific utilization rate of nitrite in the MEC was also higher (0.3 mmol N g−1 VSS h−1) than that of Control 1 (0.2 mmol N g−1 VSS h−1), implying that the MEC accelerated the anammox reactions. A wide range (0.09 to 3.74 mmol N g−1 VSS h−1) of the maximum specific utilization rate had been reported for conventional anammox and was associated with anammox bacteria enrichment and operation modes.29 In this study, anoxic bacteria (e.g. denitrifying bacteria, electrogenic bacteria) in the MEC co-present with anammox bacteria, which may result in a maximum specific utilization rate (0.3 to 0.38 mmol N g−1 VSS h−1) that is not higher than the reported values. Additionally, a high specific utilization rate was reported in continuous flow mode with high N-loading (concentration: 230 mg-N L−1);28 meanwhile, the MEC was operated in batch mode in this study, with a total nitrogen concentration of 90 mg-N L−1. Nevertheless, the ammonium utilization rate in the MEC (anammox with power supply) was twice as in Control 1 (conventional anammox) in this study, indicating the substantial acceleration of anammox in MECs.
Table 1 Values of kinetic parameters derived from kinetics models (eqn (1) to (4))
Symbol |
Description |
Value |
Unit |
K
SNH4+MEC
|
Half-velocity constant of ammonium in anammox MEC |
4.11 |
mM |
K
SNO2−MEC
|
Half-velocity constant of nitrite in anammox MEC |
2.33 |
mM |
K
SNH4+ana
|
Half-velocity constant of ammonium in Control 1 |
3.47 |
mM |
K
SNO2−ana
|
Half-velocity constant of nitrite in Control 1 |
3.4 |
mM |
μ
mNH4+MEC
|
Maximum specific utilization rate of ammonium in anammox MEC |
0.38 |
mmol g−1 VSS h−1 |
μ
mNO2−MEC
|
Maximum specific utilization rate of nitrite in anammox MEC |
0.3 |
mmol g−1 VSS h−1 |
μ
mNH4+ana
|
Maximum specific utilization rate of ammonium in Control 1 |
0.18 |
mmol g−1 VSS h−1 |
μ
mNO2−ana
|
Maximum specific utilization rate of nitrite in Control 1 |
0.2 |
mmol g−1 VSS h−1 |
As for the half-velocity constant (KS), a wide range (0.003 to 13.7 mM) has been obtained from various model simulations.26–29KS for ammonium (3.47 mM) and nitrite (3.40 mM) were almost the same in Control 1 (conventional anammox) (Table 1), which corresponds to the equal consumption of ammonium and nitrite in the anammox reaction (NH4+ + NO2− → N2 + 2H2O). In contrast, the nitrite constant (2.33 mM) was lower than the ammonium constant (4.11 mM) in the anammox MEC (Table 1), indicating that ammonium was more easily utilized than nitrite; thus, the power supply of MECs promotes ammonium utilization over that of nitrite. These results corresponded well with the results of the removal rates of ammonium and nitrite in the anammox MEC and Control 1 (Fig. 3), implying that the power supply of the MEC assisted the ammonium uptake more than nitrite.
Variation of ORP and pH throughout the nitrogen removal processes
ORP (redox potential) and pH are critical indicators for BNR processes.1,52 The ORP values increased over time in the anammox MEC, Control 1 and Control 2 (fed with nitrite), but decreased greatly in Control 2 (fed with ammonium) and remained stable in Control 3 (Fig. 5b). For Control 2, which had ammonium input, there was no oxidizer in the system; therefore, the ORP dropped as anoxic bacteria consumed the residue while oxygen dissolution and fermentation occurred, which also explains the low ammonium removal rate (Fig. 3). For Control 3 (abiotic test), without anoxic bacteria, the ORP did not change, indicating that the reactions barely occur without anoxic bacteria; this further proved the function of bacteria in the anammox MECs. For the MEC test, Control 1 and Control 2 (fed with nitrite), ammonium was oxidized with nitrite as the electron acceptor, and the ORPs increased over time.
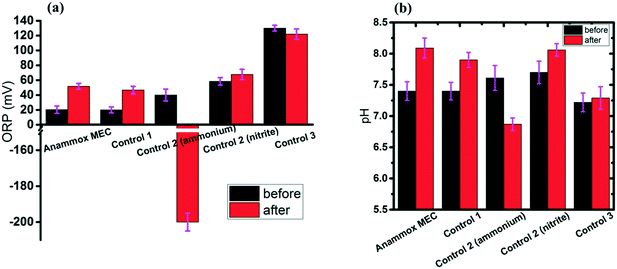 |
| Fig. 5 ORP (a) and pH (b) changes in anammox MEC and controls before and after the experiments. | |
The pH values increased in the MEC, Control 1, and Control 2 (fed with nitrite) (Fig. 5b), indicating the occurrence of denitrification, since the pH did not change in anammox (NH4+ + NO2− → N2 + 2H2O). In contrast, the pH dropped from 7.61 to 6.87 in Control 2 (fed with ammonium), which verified the occurrence of fermentation and corresponded to the ORP drop (Fig. 5a). The pH remained constant in Control 3 (abiotic test without bacteria), indicating the absence of biochemical reactions; this corresponded to the low nitrogen removal rate (Fig. 3).
The interface between the SCMFCs and the anammox MEC
The power required by the anammox MEC was provided from the treatment of wastewater by the SCMFCs. The variation of COD concentration (ΔCOD) in the SCMFCs was correlated with the targeted nitrogen concentration (ΔCN) in the anammox MEC (eqn (5) and (6)). For the anammox MEC, the amount of electrons transferred per mole of nitrogen to the anode (n in eqn (6)) was determined based on the direct donation species of nitrogen. With ammonium as the electron donor for the anode (Fig. 4), the value of n was 3 in eqn (6) because 3 moles of electrons were transferred per mole of N in NH4+ to form nitrogen gas. The simulations of eqn (5) and (6) revealed the linear relationship between the COD change in the SCMFCs and the nitrogen concentration change in the MEC (Fig. 6a).
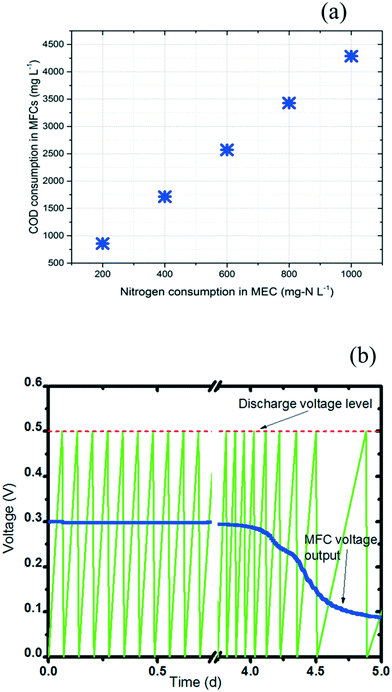 |
| Fig. 6 Interface simulation between MFC and MEC. a: Correlation between COD consumption in MFCs and nitrogen consumption in MECs. b: Charging/discharging cycles of the PMS. | |
COD consumption in the MFCs was selected in the range of 0 to 4500 mg L−1 based on diverse types of wastewater (municipal wastewater COD: 100 to 500 mg L−1, food wastewater COD: ∼8000 mg L−1).53,54 A nitrogen consumption range of 0 to 1000 mg L−1 in MECs was selected because the ammonium concentration can reach ∼1000 mg L−1 in certain types of wastewater (e.g. reject water).55 The positive linear correlation of the nitrogen and COD concentrations in the integrated MFC-MEC was different from that of conventional BNR, where the nitrogen removal deteriorated at high COD concentration due to the dominance of heterotrophic bacteria over autotrophic nitrifying bacteria.1 Meanwhile, a sufficient carbon source is needed for denitrification in conventional BNR.1 Therefore, BNR has a strict requirement for COD concentration. The integrated MFC-MEC substantially overcame this requirement because COD was consumed in the MFCs to provide electric power for the MECs; also, nitrogen removal in the MECs does not solely rely on slow-growing anammox bacteria, which greatly reduced the dependence on COD concentration. With more COD being consumed in the MFCs, higher electric power was supplied to the MECs for accelerated anammox and autotrophic denitrification.
A reliable interface between the MFC and MEC should be built using a power management system (PMS) in order to provide stable voltage from the MFCs to the MECs.22,56 In a PMS, the current rapidly passes through the MFC to charge a capacitor to a given charge potential value (Vc: 0.5 V in this study), and then the system begins to discharge the stored energy to the MEC until the capacitor reaches a discharge potential value (Vd: 0 V in this study). Throughout these charging/discharging cycles, the PMS can provide more stable voltage and power from the MFCs to the MEC than direct connection of the MFCs to the MEC. The charge and discharge curves of the PMS capacitor were simulated (Fig. 6b). With an initial COD concentration of 2000 mg L−1 in the batch-mode SCMFCs, stable charging/discharging cycles lasted for 4 days with a frequency of 0.167 mHz (estimated based on previous research56). As the COD was consumed in the batch-mode SCMFC over time, the voltage provided from the MFC started to drop after 4 days when the COD was below 500 mg L−1. The frequency slowed to 0.030 mHz at the end of the 5th day. With a PMS to connect the MFCs and MEC, the drop of power output can be alleviated by adjusting the charge/discharge frequency.
Energy savings of high-rate anammox in the integrated MFC-MEC system
The operational costs of the integrated MFC-MEC system and conventional BNR were compared in terms of energy and carbon source. The electric power consumption in conventional BNRs is around 0.505 kWh m−3, with aeration during complete nitrification as the main electricity cost.57 In contrast, anammox in the integrated MFC-MEC system only requires short-cut nitrification for nitrite production; therefore, one third of the electric power consumed by traditional BNR (∼0.189 kWh m−3) was consumed by the integrated MFC-MEC system (Table 2). Furthermore, nitrite could be produced without energy consumption. For example, nitrite can be produced in MFCs with oxygen as the electron acceptor in the cathode (2NH4+ + 3O2 → 2NO2− + 2H2O + 4H+).15 Nitrite accumulated in autotrophic denitrification MFCs without aeration (NO3− + 2H+ + 2e− → NO2− + H2O, 2NO2− + 8H+ + 6e− → N2 + 4H2O) can be used for anammox.31
Table 2 Comparison of anammox MEC, conventional anammox and BNR regarding energy consumption and saving
|
Nitrification and denitrification in conventional BNR57 |
Conventional anammox26–29 |
Anammox MEC powered by MFC |
Oxygen consumption (mole per mole of NH4+) |
2 |
0.75 |
0.75 |
Energy production (kWh m−3) |
— |
— |
0.28 |
Energy consumption (kWh m−3) |
0.505 |
0.19 |
0.19 |
Energy saving (kWh m−3) |
−0.505 |
−0.19 |
+0.09 |
COD requirement (mg L−1 per mg-N L−1) |
3.5 to 5 |
— |
— |
μ
m (mmol g−1 VSS h−1) |
1.21 to 9.86 |
0.09 to 3.74 (0.18 to 0.2 in Control 1, this study) |
0.3 to 0.38 |
Another economy of anammox compared with conventional denitrification is the carbon source, because anammox microorganisms are autotrophic.2 Nitrogen removal in the MECs did not require organic carbon, whereas in conventional BNR, a carbon source is required for heterotrophic denitrification; this COD was about 3.5 to 5.0 of NO3–N−1 (Table 2). Moreover, anammox was expedited in the MECs powered by two series-connected single-chamber MFCs (SCMFCs) in this study (Fig. 1). One MFC produced a maximum power density of 417 mW m−2 with a maximum voltage output of 0.3 V at an external resistance (Rext.) of 800 Ω. The series connection produced a steady voltage of ∼0.50 V for the MEC and provided 0.28 kWh m−3 energy for nitrogen removal over the 10 day period. Therefore, the integrated MFC-MEC system is capable of accelerating anammox with the power produced from wastewater and of achieving self-sustained high-rate nitrogen removal with low energy and carbon costs.
Significance of accelerating anammox in the integrated MFC-MEC system for wastewater treatment plants
The low growth rate of anammox bacteria presents a major obstacle for the acceptance of anammox in wastewater treatment plants. An innovative solution was explored in this study by expediting anammox in an integrated MFC-MEC system without solely relying on the traditional anammox process, thus expanding the applications of anammox. The simultaneous removal of ammonium and nitrite under anoxic conditions clearly indicated the occurrence of anammox in the MECs, given that previous anammox MFC studies with similar inoculation/operational protocols showed the same ammonium/nitrite trend as this study and confirmed the existence of anammox bacteria.39–42 By supporting MECs with electric power generated in MFCs by wastewater treatment, the integrated system effectively utilized the unique features of MFCs (long-term power production stability but low power output) and achieved self-sustained anammox with a higher nitrogen removal rate (8.2 mg-N L−1 d−1) than conventional anammox (6.8 mg-N L−1 d−1). Although the reaction rate was limited in the batch-mode system compared with conventional continuous flow BNRs (μm value, Table 2), the integrated MFC-MEC system was more energy efficient and required much less carbon than conventional BNRs.
Conclusion
A novel integrated MEC-MFC system was developed to accelerate anammox without external power consumption and alleviate the dependence on slow-growing anammox bacteria. Batch-mode tests clearly showed that more than 85% of nitrogen was removed in the anammox MEC within 10 days, while only 62% of nitrogen was removed by conventional anammox. Simulation of the modified Nernst–Monod model revealed enhancements of the half-velocity constant (Ks) and the maximum specific utilization rate (μm) in the anammox MFC over conventional anammox. The power supply of the MECs enabled electron transfer from ammonium to the anode, leading to a higher anammox rate. MFCs as the power supply to the MECs achieved self-sustained wastewater treatment while effectively utilizing the low power output of MFCs. By lowering energy consumption, avoiding carbon requirements, and accelerating anammox in a single unit, the integrated MFC-MEC system possesses great potential for self-sustained advanced nitrogen removal in wastewater treatment plants.
Acknowledgements
The graduate student (Yan Li) is supported by the China Scholarship Council (CSC) Doctorate Program.
References
-
G. Tchobanoglous, F. L. Burton and H. D. Stensel, Wastewater engineering, treatment and reuse, Metcalf&Eddy, New York, 4th edn, 2003 Search PubMed.
- M. Strous, E. V. Gerven, P. Zheng, J. G. Kuenen and M. S. M. Jetten, Water Res., 1997, 31, 1955–1962 CrossRef CAS.
- S. Q. Ni and J. Zhang, BioMed. Res. Int., 2013, 469360, DOI:10.1155/2013/469360.
- R. A. Rozendal, H. V. Hamelers and C. J. Buisman, Environ. Sci. Technol., 2006, 40, 5206–5211 CrossRef CAS PubMed.
- D. Pant, G. V. Bogaert, L. Diels and K. Vanbroekhoven, Bioresour. Technol., 2010, 101, 1533–1543 CrossRef CAS PubMed.
- U. Schröder, F. Harnisch and L. T. Angenent, Energy Environ. Sci., 2015, 8, 513–519 Search PubMed.
- M. R. Arredondo, P. Kuntke, A. W. Jeremiasse, T. H. J. A. Sleutels, C. J. N. Buisman and A. ter Heijne, Environ. Sci.: Water Res. Technol., 2015, 1, 22–33 Search PubMed.
- B. Xu, Z. Ge and Z. He, Environ. Sci.: Water Res. Technol., 2015, 1, 279–284 CAS.
- C. Santoro, I. Ieropoulos, J. Greenman, P. Cristiani, T. Vadas, A. Mackay and B. Li, J. Power Sources, 2013, 238, 190–196 CrossRef CAS.
- C. Santoro, A. Stadlhofer, V. Hacker, G. Squadrito, U. Schröder and B. Li, J. Power Sources, 2013, 243, 499–507 CrossRef CAS.
- Y. Li, Y. Wu, S. Puranik, Y. Lei, T. Vadas and B. Li, J. Power Sources, 2014, 269, 430–439 CrossRef CAS.
- B. E. Logan, B. Hamelers, R. Rozendal, U. Schröder, J. Keller, S. Freguia, P. Aelterman, W. Verstraete and K. Rabaey, Environ. Sci. Technol., 2006, 40, 5181–5192 CrossRef CAS PubMed.
- Y. Li, Y. Wu, B. Liu, H. Luan, T. Vadas, W. Guo, J. Ding and B. Li, Bioresour. Technol., 2015, 192, 238–246 CrossRef CAS PubMed.
- F. Zhang and Z. He, J. Chem. Technol. Biotechnol., 2012, 87, 153–159 CrossRef CAS.
- B. Virdis, K. Rabaey, R. A. Rozendal, Z. Yuan and J. Keller, Water Res., 2010, 44, 2970–2980 CrossRef CAS PubMed.
- Y. Li, I. Williams, Z. Xu, B. Li and B. Li, Appl. Energy, 2016, 163, 352–360 CrossRef CAS.
- G. Zhan, L. Zhang, D. Li, W. Su, Y. Tao and J. Qian, Bioresour. Technol., 2012, 116, 271–277 CrossRef CAS PubMed.
- L. Hang, X. Chai, G. Chen and B. E. Logan, Environ. Sci. Technol., 2011, 45, 5025–5031 CrossRef PubMed.
- Z. He, J. Kan, Y. Wang, Y. Huang, F. Mansfield and K. H. Nealson, Environ. Sci. Technol., 2009, 43, 3391–3397 CrossRef CAS PubMed.
- X. Xie, M. Ye, L. Hu, N. Liu, J. R. McDonough, W. Chen, H. N. Alshareef, C. S. Criddle and Y. Cui, Energy Environ. Sci., 2012, 5, 5265–5270 CAS.
- Y. Chen, Z. Lv, J. Xu, D. Peng, Y. Liu, J. Chen, X. Sun, C. Feng and C. Wei, J. Power Sources, 2012, 201, 136–141 CrossRef CAS.
- B. Liu, A. Weinstein, M. Kolln, C. Garrett, L. Wang, A. Bagtzoglou, U. Karra, Y. Li and B. Li, J. Power Sources, 2015, 286, 210–216 CrossRef CAS.
- Z. Xu, B. Liu, Q. Dong, Y. Lei, Y. Li, J. Ren, J. McCutcheon and B. Li, Bioresour. Technol., 2015, 197, 244–251 CrossRef CAS PubMed.
- M. C. Hatzell, Y. Kim and B. E. Logan, J. Power Sources, 2013, 229, 198–202 CrossRef CAS.
- M. E. Nielsen, C. E. Reimers, H. K. White, S. Sharma and P. R. Girguis, Energy Environ. Sci., 2008, 1, 584–593 CAS.
- S. Q. Ni, S. Sung, Q. Y. Yue and B. Y. Gao, Ecol. Eng., 2012, 38, 30–36 CrossRef.
- M. Strous, J. J. Heijnen, J. G. Kuenen and M. S. M. Jetten, Appl. Microbiol. Biotechnol., 1998, 50, 589–596 CrossRef CAS.
- T. Chen, P. Zheng, L. Shen, S. Ding and Q. Mahmood, J. Hazard. Mater., 2011, 190, 28–35 CrossRef CAS PubMed.
- D. Puyol, J. M. Carvajal-Arroyo, B. Garcia, R. S. Alvarez and J. A. Field, Bioresour. Technol., 2013, 139, 94–100 CrossRef CAS PubMed.
- A. K. Marcus, C. I. Torres and B. E. Rittmann, Biotechnol. Bioeng., 2007, 98, 1171–1182 CrossRef CAS PubMed.
- V. Srinivasan, J. Weinrich and C. Butler, Environ. Sci.: Water Res. Technol., 2016, 2, 344–352 CAS.
- H. Liu, S. Cheng and B. E. Logan, Environ. Sci. Technol., 2005, 39, 658–662 CrossRef CAS PubMed.
- B. Virdis, K. Rabaey and J. Yuan, Water Res., 2008, 42, 3013–3024 CrossRef CAS PubMed.
- S. Xie, P. Liang, Y. Chen, X. Xia and X. Huang, Bioresour. Technol., 2011, 102, 348–354 CrossRef CAS PubMed.
-
L. K. Wang, N. C. Pereira, Y. T. Huang and N. K. Shammas, Biological treatment processes, Humana Press, New York, vol. 8, 2009 Search PubMed.
- M. S. M. Jetten, M. Strous, K. T. van de Pas-Schoonen, J. Schalk, U. G. J. M. van Dongen, A. A. van de Graaf, S. Logemann, G. Muyzer, M. C. M. van Loosdrecht and J. G. Kuenen, FEMS Microbiol. Rev., 1999, 22, 421–437 CrossRef.
- R. Zhao, H. Zhang, Y. Li, T. Jiang and F. Yang, Curr. Microbiol., 2014, 69, 880–887 CrossRef CAS PubMed.
- M. Oshiki, I. K. Yoshida, N. Fujii, M. Ishiguro, H. Satoh and S. Okabe, Appl. Environ. Microbiol., 2013, 79, 4087–4093 CrossRef CAS PubMed.
- Y. Lee, L. Martin, P. Grasel, K. Tawfiq and G. Chen, Environ. Technol., 2013, 34, 2727–2736 CrossRef CAS PubMed.
- E. G. Di Domenico, G. Petroni, D. Mancini, A. Geri, L. Di Palma and F. Ascenzioni, BioMed. Res. Int., 2015, 351014, DOI:10.1155/2015/351014.
- C. Li, H. Ren, M. Xu and J. Cao, Bioresour. Technol., 2015, 175, 454–552 CrossRef PubMed.
- D. A. Jadhav and M. M. Ghangrekar, Environ. Technol., 2015, 36, 767–775 CrossRef CAS PubMed.
- M. K. H. Winkler, R. Kleerebezem and M. C. M. van Loosdrecht, Water Res., 2012, 46, 136–144 CrossRef CAS PubMed.
- T. Yamamoto, K. Takaki, T. Koyama and K. Furukawa, Bioresour. Technol., 2008, 99, 6419–6425 CrossRef CAS PubMed.
- X. Xu, Y. Xue, D. Wang, G. Wang and F. Yang, Bioresour. Technol., 2014, 155, 427–431 CrossRef CAS PubMed.
- E. M. Gilbert, S. Agrawal, S. M. Karst, H. Horn, P. H. Nelsen and S. Lackner, Environ. Sci. Technol., 2014, 48, 8784–8792 CrossRef CAS PubMed.
- C. Tang, P. Zheng, C. Wang, Q. Mahmood, J. Zhang, X. Chen, L. Zhang and J. Chen, Water Res., 2011, 45, 135–144 CrossRef CAS PubMed.
- W. R. L. van der Star, W. R. Abma, D. Blommers, J. Mulder, T. Tokutomi, M. Strous, C. Picioreanu and M. C. M. van Loosdrecht, Water Res., 2007, 41, 4149–4163 CrossRef CAS PubMed.
- M. Waki, T. Yasuda, K. Suzuki, T. Sakai, N. Suzuki, R. Suzuki, K. Matsuba, H. Yokoyama, A. Ogino, Y. Tanaka, S. Ueda, M. Takeuchi, T. Yamagishi and Y. Suwa, Bioresour. Technol., 2010, 101, 2685–2690 CrossRef CAS PubMed.
- B. Kartal, M. Koleva, R. Arsov, W. van der Star, M. S. M. Jetten and M. Strous, J. Biotechnol., 2006, 126, 546–553 CrossRef CAS PubMed.
- K. Egli, U. Fanger, P. J. J. Alvarez, H. Siegrist, J. R. van der Meer and A. J. B. Zehnder, Arch. Microbiol., 2001, 175, 198–207 CrossRef CAS PubMed.
- B. Li and S. Irvin, Biochem. Eng. J., 2007, 34, 248–255 CrossRef CAS.
- J. Ditzig, H. Liu and B. E. Logan, Water Res., 2007, 32, 2296–2304 CAS.
- S. E. Oh and B. E. Logan, Water Res., 2005, 39, 4673–4682 CrossRef CAS PubMed.
- P. T. Kelly and Z. He, Bioresour. Technol., 2014, 153, 351–360 CrossRef CAS PubMed.
- A. Dewan, C. Donovan, D. Heo and H. Beyenal, J. Power Sources, 2010, 195, 90–96 CrossRef CAS.
-
R. Goldstein and W. Smith, Water & sustainability (volume 4): U.S. electricity consumption for water supply & treatment-the next half century, Electric power research institute, Incn, Palo Alto, 2002 Search PubMed.
|
This journal is © The Royal Society of Chemistry 2016 |
Click here to see how this site uses Cookies. View our privacy policy here.