DOI:
10.1039/C5SC01545F
(Edge Article)
Chem. Sci., 2015,
6, 5059-5062
Well-defined aqueous nanoassemblies from amphiphilic meta-terphenyls and their guest incorporation†
Received
28th April 2015
, Accepted 8th June 2015
First published on 9th June 2015
Abstract
Spherical molecular assemblies with diameters of ∼2 nm were quantitatively formed in water from new amphiphilic meta-terphenyls with two hydrophilic pendants at the central benzene ring. Whereas intermolecular interactions between small aromatic rings are typically weak, the obtained nanoassemblies are stable enough at wide-ranging concentrations and mostly remain intact even in the presence of similar nanoassemblies with polyaromatic frameworks. The nanoassembly with pentamethyl-substituted terminal benzene rings provides superior host capability for fluorescent dyes in water.
Introduction
A benzene ring, the minimal unit of polyaromatic hydrocarbons, is widely utilized for the construction of various molecular architectures.1,2 However, intermolecular interactions between such small aromatic rings are relatively weak as compared with those between extended polyaromatic rings (e.g., anthracene and pyrene) therefore the certain formation of well-defined, benzene-based molecular assemblies requires core frameworks containing multiple benzene rings linked covalently.3,4 For instance, Lee et al. reported that linear amphiphilic compounds with a quaterphenyl framework (C6H5–C6H4–C6H4–C6H5) generate large micellar or vesicle-like assemblies (>∼20 nm in diameter) in water.5 The bent oligophenyl (C6H5–(C6H4)7–C6H5) amphiphiles also form huge tubular assemblies.5 On the other hand, small frameworks composed of two or three benzene rings have not proven useful for the subunits of discrete molecular assemblies.6
Here we employed a meta-terphenyl framework to explore new molecular assemblies with well-controlled size and shape distributions as well as guest incorporation ability. V-shaped amphiphilic compounds 1a and 1b, designed here (Fig. 1a), are composed of a meta-terphenyl skeleton with two hydrophilic pendants at the central benzene ring. To force the terminal benzene rings to adopt an orthogonal conformation with respect to the central ring, methyl groups were employed as substituents on the terminal rings (1a; Fig. 1b).7 In this context, we have recently revealed that a similar amphiphilic molecule 1c with a bent meta-di(anthryl)benzene framework generates a spherical polyaromatic assembly 2c in water through effective π-stacking interactions.8 Herein, we report the quantitative formation of well-defined, spherical assemblies 2a and 2b from ∼5 molecules of 1a and 1b in aqueous media. The pentamethylbenzene-shelled assembly 2a effectively incorporates well-known fluorescent dyes (i.e., fluorescein and Eosin Y) in water at room temperature and the emissive colors of the encapsulated dyes are altered by the host–guest interactions.
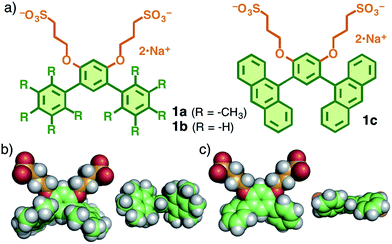 |
| Fig. 1 (a) V-shaped amphiphilic compounds 1a–c. The optimized structures of (b) 1a and (c) 1b (side and front views) without the counterions, obtained by DFT calculations (B3LYP/6-31G(d,p) level). | |
Results and discussion
Quantitative formation of the spherical nanoassemblies
V-shaped amphiphilic compound 1a was synthesized through four step reactions with ∼35% overall yield.9 Once a white solid of 1a (2.0 μmol) was dissolved in water (1.0 mL) at room temperature, spherical assembly 2a was quantitatively formed within 1 min (Fig. 2a). The 1H NMR spectrum of 2a in D2O is similar to that of 1a in CD3OD and contains two aromatic (Ha,b) and six aliphatic (Hc–h) signals (Fig. 2b and c). On the other hand, the observed diffusion coefficients (D) of 2a in D2O and 1a in CD3OD were evidently different. The diffusion-ordered spectroscopy (DOSY) NMR spectrum of 2a displayed a single band at a D of 4.3 × 10−10 m2 s−1 (Fig. 2e), which is smaller than that of 1a (8.3 × 10−10 m2 s−1; Fig. 2d). On the basis of the D value and the Stokes–Einstein equation, the core diameter of 2a was calculated to be about 1 nm. Further structural evidence for 2a was obtained by atomic force microscopy (AFM). We observed uniform, spherical particles with an average height of 2.2 nm on the AFM image (Fig. 2f), prepared by drop casting from an aqueous solution of 2a (1.0 mM based on 1a) on a mica surface. The diameter of the spherical assembly of pentamer (1a)5 including the outer sulfonate groups (2.5 nm)10 is comparable to that of 2a obtained by the AFM analysis (Fig. 2g).
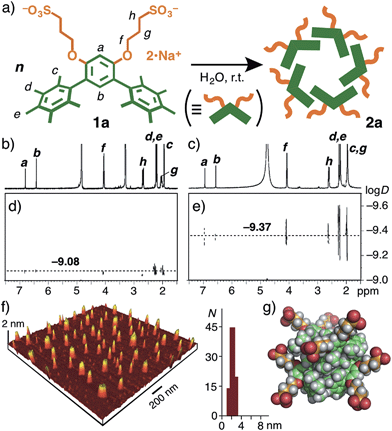 |
| Fig. 2 (a) Schematic representation of the formation of spherical nanoassembly 2a from V-shaped amphiphilic compound 1a in water. 1H NMR and DOSY spectra (400 MHz, 25 °C) of (b and d) 1a in CD3OD and (c and e) 2a in D2O (2.0 mM based on 1a). (f) AFM image of 2a on mica and the size and number (N) distribution. (g) Molecular modeling of 2a, composed of five molecules of 1a. | |
It is worth noting that the methyl groups on the terminal benzene rings of 1a are not essential for the formation of well-defined, small nanoassemblies. Thus, in a manner similar to 2a, simple V-shaped amphiphile 1b without the methyl groups7 quantitatively formed spherical assembly 2b in water at room temperature. The size of the product is slightly smaller than that of 2a but the shape is quite similar to that of 2a, as confirmed by DOSY NMR, DLS, and AFM analyses (Fig. S38–41†).9 In agreement with our previous study of amphiphile 1c with a bent polyaromatic framework,8 the meta-terphenyl moiety was therefore proved to be one of the key skeletons in this supramolecular system.
Photophysical properties and stability of the spherical nanoassemblies
Assemblies 2a and 2b turned out to be stable enough in water under ambient conditions. Dynamic light scattering (DLS) analysis of 2a indicated the exclusive existence of small particles (dav. = 1.6 nm) with a sharp size distribution (±1 nm) at the monomer concentration of 1.0 mM. Concentration-dependent DLS studies revealed that the particles of 2a and 2b remained intact at up to 10 mM in water at room temperature for more than one week (Fig. S39 and 40†). The water-solubility of 2a and 2b is higher than that of 2c (up to ∼2 mM based on 1c). Concentration-dependent fluorescence studies (in the range of 0.001–1.0 mM based on 1a) elucidated that the critical micelle concentration (CMC) value of 2a is ∼0.005 mM.
The UV-visible spectra of monomer 1a in methanol and assembly 2a in water exhibited similar absorption bands at λmax = ∼290 nm (Fig. 3a). The absorption bands are blue-shifted (Δλ = ∼10 nm) as compared with those of 1b and 2b. Similarly, the emission bands of 1a and 2a are also blue-shifted (Δλmax = ∼30 nm) with respect to those of 1b and 2b (Fig. 3b). These photophysical properties can be explained by the conformational difference between the two amphiphiles. Sterically hindered 1a adopts a rigidly bent structure for the methyl-substituted meta-terphenyl moiety but 1b adopts a slightly twisted, planar structure for the meta-terphenyl framework with an extended π-conjugated system, as also suggested by the optimized structures of 1a and 1b (Fig. 1b and c).
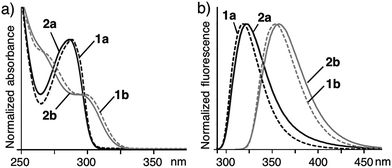 |
| Fig. 3 (a) Normalized UV-visible and (b) fluorescence spectra (r.t.) of nanoassemblies 2a and 2b in H2O (1.0 mM based on 1a and 1b) and monomers 1a and 1b in CH3OH. Excitation wavelengths: λex = 286 nm for 1a and 2a, λex = 299 nm for 1b and 2b. | |
Mixing two linear amphiphilic compounds with different hydrophobic alkyl chains usually generates a complex mixture of spherical aggregates with a statistical distribution of the amphiphiles in water.11 In contrast, the combination of V-shaped amphiphiles 1a and 1c led to an equilibrium mixture of nanoassemblies, mainly including 2a and 2c (Fig. 4a). When 1a and 1c (in a 1
:
1 ratio) were agitated in H2O (1.0 mM) at room temperature for 1 min, the resultant solution was analyzed by UV-visible and fluorescence spectroscopies. The observed absorption and emission bands nearly overlapped with the sum of the bands of assemblies 2a and 2c (Fig. 4b and c). No coalesced emission bands were observed, in contrast to similar polyaromatic assemblies (Fig. S47 and 48†).9 In addition, the mixed solution of 2a and 2c in H2O at room temperature (even after heating at 80 °C for 10 min) exhibited the same spectrum. These behaviors are most probably caused by the difference of the major intermolecular interactions between the two amphiphiles: 2a forms through hydrophobic effects but 2c forms through π-stacking interactions in aqueous solutions.
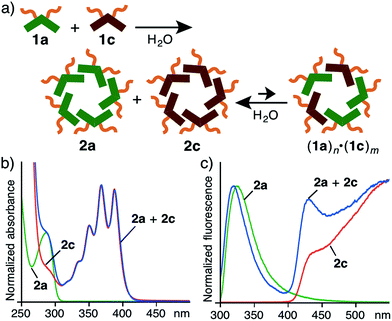 |
| Fig. 4 (a) Schematic representation of the selective formation of nanoassemblies 2a and 2c from a mixture of 1a and 1c in water. (b) Normalized UV-visible and (c) fluorescence spectra (H2O, r.t., λex = 286 nm) of 2a, 2c, and 2a + 2c (1.0 mM based on 1a and 1c each). | |
Effective incorporation of fluorescent dyes
Surprisingly, the guest incorporation ability of 2a and 2b is strikingly different in water. Nanoassembly 2a with its bent bis-pentamethylbenzene frameworks promoted guest incorporation. For example, an excess amount of fluorescein (3; 1.0 μmol) with slightly hydrophilic properties was suspended in a D2O solution (2.0 mL) of 2a (2.0 μmol based on 1a) and the resultant mixture was stirred at room temperature for 1 h (Fig. 5a). The filtration of the suspended mixture yielded 2a·(3)n as a clear pale-yellow solution. In the 1H NMR spectrum, new signals derived from encapsulated 3 were clearly observed in the range of 6.6–7.9 ppm (Fig. 5b), whereas the proton signals of free 3 were hardly detectable in water (Fig. S50†). The UV-visible spectrum showed a new prominent absorption band at λmax = 487 nm assignable to the incorporated guest molecules (Fig. 5c). In stark contrast, similar assemblies 2b and 2c exhibited weak binding abilities for 3 under the same conditions, as revealed by the UV-visible analysis (Fig. 5c).
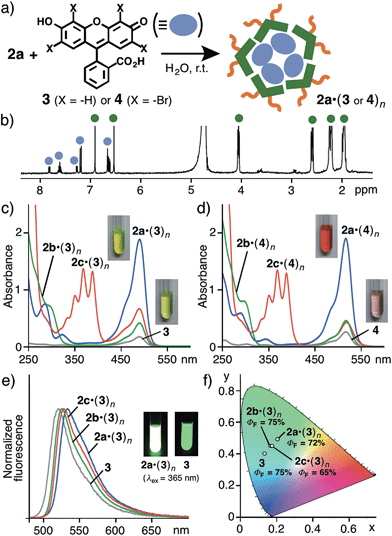 |
| Fig. 5 (a) Schematic representation of the incorporation of fluorescein (3) or Eosin Y (4) by nanoassembly 2a in water. (b) 1H NMR spectrum (400 MHz, r.t.) of 2a·(3)n in D2O. UV-visible spectra (r.t. H2O) of (c) nanoassemblies 2a–c·(3)n and (d) 2a–c·(4)n (1.0 mM based on 1a–c). (e) Normalized fluorescence spectra (r.t. H2O, λex = 487 nm) and (f) CIE coordinate diagram and quantum yields of 3 and 2a–c·(3)n. | |
Eosin Y (4) was also effectively incorporated into 2a in water at room temperature to give a red solution due to the formation of 2a·(4)n (Fig. 5d).9,12 Assemblies 2b and 2c showed lower binding abilities for 4. On the basis of the absorption intensities, the concentrations of 3 and 4 in the obtained aqueous solutions of 2a·(3)n and 2a·(4)n were estimated to be 0.32 and 0.30 mM, respectively.13 The values are >20-times larger than those of the saturated solutions of 3 and 4 in water without 2a–c. Furthermore, the intense fluorescence band (ΦF = 72%) of the incorporated 3 within 2a was found at λmax = 532 nm, which is slightly red-shifted (Δλmax = 13 nm) as compared with that of free 3 due to being enclosed in the pentamethylbenzene frameworks (Fig. 5e). The difference in the total emission colors of 3 and 2a–c·(3)n is clearly revealed in the CIE chromaticity diagram (Fig. 5f). Thus, it is revealed that the bent conformation of the pentamethyl-substituted meta-terphenyl framework is of importance for the formation of a nanoassembly with an inner space capable of binding guest molecules.
Conclusions
In conclusion, we have revealed that meta-terphenyl, a small benzene oligomer, acts as a useful building block for the quantitative formation of well-defined, spherical assemblies (∼2 nm in diameter) in water by attaching hydrophilic groups to the central benzene ring. The nanoassemblies are stable enough in a wide range of concentrations and remain intact even when mixed with a similar nanoassembly with polyaromatic frameworks. Moreover, pentamethyl substitution on the terminal benzene rings of the amphiphile forces the meta-terphenyl framework to adopt a bent conformation, which imparts the nanoassembly with a superior hosting capability for fluorescent dyes in water. The present study demonstrated the utility of a simple C6H5–C6H4–C6H5 skeleton for discrete supramolecular systems with designed structures and functions.
Acknowledgements
This work was supported by the Japan Society for the Promotion of Science (JSPS) through a “Funding Program for Next-Generation World-Leading Researchers (NEXT Program)” and by the Japanese Ministry of Education, Culture, Sports, Science and Technology (MEXT) through Grants-in-Aid for Scientific Research on Innovative Areas “Soft Molecular Systems”. K.K. thanks JSPS for a Research Fellowship for Young Scientists.
Notes and references
-
(a) K. N. Baker, A. V. Fratini, T. Resch, H. C. Knachel, W. W. Adams, E. P. Socci and B. L. Farmer, Polymer, 1993, 34, 1571–1587 CrossRef CAS;
(b) D. J. Williams, H. M. Colquhoun and C. A. O'Mahoneya, Chem. Commun., 1994, 1643–1644 RSC;
(c) M. Panda and J. Chandrasekhar, J. Am. Chem. Soc., 1998, 120, 13517–13518 CrossRef CAS;
(d) N. Kobayashi, S. Sasaki, M. Abe, S. Watanabe, H. Fukumoto and T. Yamamoto, Macromolecules, 2004, 37, 7986–7991 CrossRef CAS.
-
(a) H. A. Staab and F. Binnig, Tetrahedron Lett., 1964, 5, 319–321 CrossRef;
(b) Y. Fujioka, Bull. Chem. Soc. Jpn., 1984, 57, 3494–3506 CrossRef CAS;
(c) C. Grave and A. D. Schlüter, Eur. J. Org. Chem., 2002, 3075–3098 CrossRef CAS;
(d) M. Iyoda, M. J. Rahman, A. Matsumoto, M. Wu, Y. Kuwatani, K. Nakao and Y. Miyake, Chem. Lett., 2005, 34, 1474–1475 CrossRef CAS;
(e) R. Jasti, J. Bhattacharjee, J. B. Neaton and C. R. Bertozzi, J. Am. Chem. Soc., 2008, 130, 17646–17647 CrossRef CAS PubMed;
(f) K. Matsui, Y. Segawa, T. Namikawa, K. Kamada and K. Itami, Chem. Sci., 2013, 4, 84–88 RSC;
(g) A. Yagi, Y. Segawa and K. Itami, J. Am. Chem. Soc., 2012, 134, 2962–2965 CrossRef CAS PubMed;
(h) E. Kayahara, T. Iwamoto, H. Takaya, T. Suzuki, M. Fujitsuka, T. Majima, N. Yasuda, N. Matsuyama, S. Seki and S. Yamago, Nat. Commun., 2013, 4, 2694 Search PubMed.
-
(a) M. Lee, C.-J. Jang and J.-H. Ryu, J. Am. Chem. Soc., 2004, 126, 8082–8083 CrossRef CAS PubMed;
(b) H.-J. Kim, T. Kim and M. Lee, Acc. Chem. Res., 2011, 44, 72–82 CrossRef CAS PubMed.
-
(a) S. Hiraoka, K. Harano, M. Shiro and M. Shionoya, J. Am. Chem. Soc., 2008, 130, 14368–14369 CrossRef CAS PubMed;
(b) S. Hiraoka, T. Nakamura, M. Shiro and M. Shionoya, J. Am. Chem. Soc., 2010, 132, 13223–13225 CrossRef CAS PubMed.
-
(a) B.-S. Kim, D.-J. Hong, J. Bae and M. Lee, J. Am. Chem. Soc., 2005, 127, 16333–16337 CrossRef CAS PubMed;
(b) Z. Huang, S.-K. Kang, M. Banno, T. Yamaguchi, D. Lee, C. Seok, E. Yashima and M. Lee, Science, 2012, 337, 1521–1526 CrossRef CAS PubMed.
-
(a) S. Leininger, B. Olenyuk and P. J. Stang, Chem. Rev., 2000, 100, 853–908 CrossRef CAS PubMed;
(b) F. Hof, S. L. Craig, C. Nuckolls and J. Rebek Jr, Angew. Chem., Int. Ed., 2002, 41, 1488–1508 CrossRef CAS;
(c) D. M. Vriezema, M. C. Aragonès, J. A. A. W. Elemans, J. J. L. M. Cornelissen, A. E. Rowan and R. J. M. Nolte, Chem. Rev., 2005, 105, 1445–1489 CrossRef CAS PubMed;
(d) J. Rebek Jr, Angew. Chem., Int. Ed., 2005, 44, 2068–2078 CrossRef PubMed;
(e) M. Fujita, M. Tominaga, A. Hori and B. Therrien, Acc. Chem. Res., 2005, 38, 371–380 CrossRef PubMed;
(f) M. D. Pluth, R. G. Bergman and K. N. Raymond, Acc. Chem. Res., 2009, 42, 1650–1659 CrossRef CAS PubMed;
(g) M. Yoshizawa, J. K. Klosterman and M. Fujita, Angew. Chem., Int. Ed., 2009, 48, 3418–3438 CrossRef CAS PubMed;
(h) M. M. Safont-Sempere, G. Fernámdez and F. Würthner, Chem. Rev., 2011, 111, 5784–5814 CrossRef CAS PubMed;
(i) R. Chakrabarty, P. Sarath Mukherjee and P. J. Stang, Chem. Rev., 2011, 111, 6810–6918 CrossRef CAS PubMed;
(j) H. Amouri, C. Desmarets and J. Moussa, Chem. Rev., 2012, 112, 2015–2041 CrossRef CAS PubMed;
(k) M. M. J. Smulders, I. A. Riddell, C. Browne and J. R. Nitschke, Chem. Soc. Rev., 2013, 42, 1728–1754 RSC;
(l) K. Harris, D. Fujita and M. Fujita, Chem. Commun., 2013, 49, 6703–6712 RSC;
(m) G. Zhang and M. Mastalerz, Chem. Soc. Rev., 2014, 43, 1934–1947 RSC;
(n) M. Han, D. M. Engelhard and G. H. Clever, Chem. Soc. Rev., 2014, 43, 1848–1860 RSC;
(o) L. Xu, L.-J. Chen and H.-B. Yang, Chem. Commun., 2014, 50, 5156–5170 RSC;
(p) A. M. Castilla, W. J. Ramsay and J. R. Nitschke, Acc. Chem. Res., 2014, 47, 2063–2073 CrossRef CAS PubMed.
- The dihedral angles between the adjacent aromatic rings of 1a and 1b are 72.7° and 40.5°, respectively (Fig. 1b and c).
-
(a) K. Kondo, A. Suzuki, M. Akita and M. Yoshizawa, Angew. Chem., Int. Ed., 2013, 52, 2308–2312 CrossRef CAS PubMed;
(b) A. Suzuki, K. Kondo, M. Akita and M. Yoshizawa, Angew. Chem., Int. Ed., 2013, 52, 8120–8123 CrossRef CAS PubMed;
(c) K. Kondo, A. Suzuki, M. Akita and M. Yoshizawa, Eur. J. Org. Chem., 2014, 33, 7389–7394 CrossRef;
(d) Y. Okazawa, K. Kondo, M. Akita and M. Yoshizawa, J. Am. Chem. Soc., 2015, 137, 98–101 CrossRef CAS PubMed;
(e) K. Kondo, M. Akita, T. Nakagawa, Y. Matsuo and M. Yoshizawa, Chem.–Eur. J., 2015, 21 CAS , in press.
- See the ESI.†.
- Molecular modeling study was carried out by using Materials Studio (version 5.0, Accelrys Software Inc., San Diego, CA). The outer diameters of the optimized structures of spherical assemblies (1a)4 and (1a)6 were estimated to be ∼2.0 and ∼3.0 nm, respectively (Fig. S49†).
-
(a)
Y. Moroi, Micelles: Theoretical and applied aspects, Plenum, New York, 1992 Search PubMed;
(b) S. P. Moulik, M. E. Haque, P. K. Jana and A. R. Das, J. Phys. Chem., 1996, 100, 701–708 CrossRef CAS;
(c) E. Lee, J.-K. Kim and M. Lee, J. Am. Chem. Soc., 2009, 131, 18242–18243 CrossRef CAS PubMed;
(d) S. Honda, T. Yamamoto and Y. Tezuka, J. Am. Chem. Soc., 2010, 132, 10251–10253 CrossRef CAS PubMed.
- The DLS analysis (H2O, r.t.) of 2a·(3)n and 2a·(4)n indicated the formation of larger particles with average diameters of 10.2 and 12.7 nm, respectively (Fig. S46†). The host-guest ratio of 2a·(3)n was estimated to be 1a
:
3 = 3
:
1.
-
(a) N. O. Mchedlov-Petrossyan and V. N. Kleshchevnikova, J. Chem. Soc., Faraday Trans., 1994, 90, 629–640 RSC;
(b) E. M. Arbeloa, G. V. Porcal, S. G. Bertolotti and C. M. Previtali, J. Photochem. Photobiol., A, 2013, 252, 31–36 CrossRef CAS.
Footnote |
† Electronic supplementary information (ESI) available: Experimental procedures and physical data. See DOI: 10.1039/c5sc01545f |
|
This journal is © The Royal Society of Chemistry 2015 |