DOI:
10.1039/C5SC00213C
(Edge Article)
Chem. Sci., 2015,
6, 3466-3470
Stable porphyrin Zr and Hf metal–organic frameworks featuring 2.5 nm cages: high surface areas, SCSC transformations and catalyses†
Received
20th January 2015
, Accepted 31st March 2015
First published on 31st March 2015
Abstract
Two isostructural porphyrin Zr and Hf metal–organic frameworks (FJI-H6 and FJI-H7) are rationally synthesized, and are constructed from 2.5 nm cubic cages. Notably, they both possess high water and chemical stability and can undergo single-crystal to single-crystal transformations to embed Cu2+ ions into the open porphyrin rings. FJI-H6 has a high BET surface area of 5033 m2 g−1. Additionally, they exhibit promising catalytic abilities to convert CO2 and epoxides into cyclic carbonates at ambient conditions.
Owing to their high surface area, permanent porosity and tunable pores, metal–organic frameworks (MOFs) have been used for catalysis,1 gas separation and storage,2 and drug delivery.3 Nonetheless, one big obstacle to the practical applications of MOFs is their stability, which includes water and chemical stability. To target stable MOFs, one effective method is the use of high-valence metal ions such as Fe3+, Al3+, Cr3+, Zr4+ and Hf4+ ions as the metal nodes.4 Compared with the traditional Cu2+, Zn2+ and Co2+ ions,5 the aforementioned trivalent or tetravalent metal ions will form stronger bonds with carboxylate groups according to the theory of hard and soft acids and bases. Therefore, the stability of the obtained frameworks will be strengthened. Furthermore, these high-valence metal ions tend to form highly connected inorganic clusters via the OH− and/or O2− bridges, which also significantly contributes to the stability of the frameworks. As for the Zr4+ ion, it prefers to form the classical 12-connected Zr6O4(OH)4 node. When assembled with linear carboxylate ligands, 3D fcu frameworks with ordered cubic cages (known as the UiO series) can be obtained.6 These kinds of Zr-based MOF often have high water and chemical stability, and can even serve as water adsorbents.7 Up to now, extensive investigation has been carried out to tune the porosity of the UiO series of Zr-MOFs by selectively removing organic linkers8 or by functionalizing the ligands (by pre-modification or post-synthetic methods).9 However, there are several examples based on the assembly of Zr6O4(OH)4 clusters with polycarboxylic ligands such as planar tetracarboxylic acids.7b,10 In addition, compared with Zr-based MOFs, Hf-based MOFs are also rare.6f,11 Herein, we present two ultra-stable metal–organic frameworks ([Zr6O4(OH)4(H2TBPP)3]n·(solvent)x) (FJI-H6) and ([Hf6O4(OH)4(H2TBPP)3]n·(solvent)x) (FJI-H7), which are isostructural and both constructed from M6O4(OH)4(CO2)12 nodes (M = Zr, Hf) and porphyrin tetracarboxylic ligands (H6TBPP = 4′,4′′′,4′′′′′,4′′′′′′′-(porphyrin-5,10,15,20-tetrayl)tetrakis([1,1′-biphenyl]-4-carboxylic acid)). As expected, both FJI-H6 and FJI-H7 have high water and chemical stability and can undergo a single-crystal-to-single-crystal (SCSC) transformation to embed Cu2+ ions into the open porphyrin rings. Interestingly, they both feature 2.5 nm cages. Notably, FJI-H6 has a high BET surface area of 5033 m2 g−1.
Results and discussion
Syntheses and structures of porphyrin Zr and Hf MOFs
Reaction of H6TBPP with ZrCl4 or HfCl4 modulated by benzoic acid gives rise to dark red crystals of FJI-H6 or FJI-H7. Single crystal X-ray structural analysis shows that FJI-H6 and FJI-H7 are isostructural.12 Therefore, we chose FJI-H6 as the example in the following discussion. FJI-H6 crystallizes in the high symmetry space group Pm
m. In the Zr6O4(OH)4 cluster, six equivalent Zr4+ ions are in a square-antiprismatic O8 coordination environment and form a regular octahedron. In this Zr6 octahedron, the eight triangular faces are alternatively capped by four μ3-OH− and four μ3-O2− groups. Additionally, the twelve edges of the Zr6 octahedron are bridged by twelve carboxylate groups from twelve unique H2TBPP ligands. At the same time, each H2TBPP ligand, in which the peripheral four phenyl rings are coplanar with the inner porphyrin rings, links four independent Zr6O4(OH)4 clusters. Thus, a rarely seen (4,12)-connected ftw framework can be acquired.10a,11b,13FJI-H6 has two kinds of polyhedral cages, i.e. a small octahedral cage and a large cubic cage. As seen in Fig. 1, the octahedral cage is constructed from two Zr6O4(OH)4 clusters and four H2TBPP ligands, with a cavity diameter of ca. 1.5 nm. However, the cubic cage consists of eight Zr6O4(OH)4 clusters as the vertices and six H2TBPP ligands as the sides. Significantly, the diameter of the cubic cage is approximately 2.5 nm, which is larger than that in PCN-221 (2.0 nm).11b Accordingly, the available volume is 15
000 Å3. Additionally, the window of the cubic cage is 1.2 nm × 2.0 nm, which allows large organic molecules to freely get in and out.
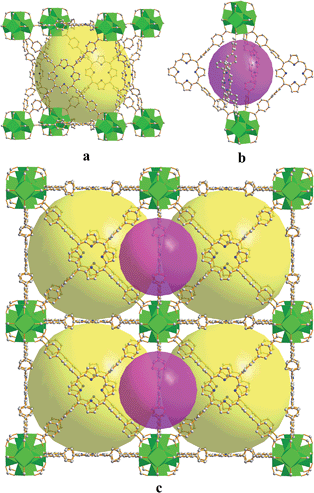 |
| Fig. 1 (a) The large cubic cage constructed from six porphyrin ligands and eight Zr6O4(OH)4 clusters. (b) The small octahedral cage constructed from four porphyrin ligands and two Zr6O4(OH)4 clusters. (c) Packing of the two kinds of cages. | |
Gas adsorption experiments and stability tests
Calculation by PLATON software14 reveals that in FJI-H6 the free volume is up to 78.6%. For FJI-H6 the permanent porosity is confirmed by an N2 adsorption isotherm measured at 77 K. The sample exchanged with acetone exhibits a reversible type I isotherm and has a saturated uptake of 1346 cm3 g−1 at 1 atm (Fig. 2). When pre-treated with 8 M HCl, the value of the N2 adsorption slightly increases to 1393 cm3 g−1, which indicates that FJI-H6 is stable with respect to the acid. From the above data, the calculated BET surface area of the sample exchanged with acetone is up to 5007 m2 g−1 (5033 m2 g−1 for the sample pre-treated with 8 M HCl), which is much larger than those of PCN-222(Fe) (2200 m2 g−1),4b NU-1000 (2320 m2 g−1),15 PCN-223(Fe) (1600 m2 g−1),10c PCN-94 (3377 m2 g−1),10b NU-1100 (4020 m2 g−1)10a and PCN-229 (4619 m2 g−1),13 but less than those of the just reported NU-1103 (5646 m2 g−1) and NU-1104 (5290 m2 g−1).10e In addition, FJI-H6 also has a high total pore volume of 2.16 cm3 g−1. The experimental BET surface area and pore volume are consistent with theoretical values calculated by Poreblazer16 (accessible surface area: 4695 m2 g−1; pore volume: 2.06 cm3 g−1),14 which demonstrates that the sample is fully activated. Additionally, FJI-H6 also shows good capacity for H2 storage. The H2 uptake reaches 172 cm3 g−1 (1.54 wt%) at 1 atm and 77 K, and 108 cm3 g−1 (0.94 wt%) at 87 K and 1 atm. Moreover, the adsorption heat of H2 calculated by the Clausius–Clapeyron equation is 6.54 kJ mol−1 at zero coverage and decreases slowly with increasing H2 loading. These values are comparable to those of famous MOF materials, such as HKUST-1 (6.6 kJ mol−1),17 MOF-5 (5.2 kJ mol−1),17 and NOTT-122 (6.0 kJ mol−1).18 As for FJI-H7 the sample exchanged with acetone also exhibits a reversible type I isotherm and has a saturated uptake of 1029 cm3 g−1 at 1 atm. From the above data, the calculated BET surface area of FJI-H7 is up to 3831 m2 g−1, which is among the highest reported for Hf-based MOFs.11
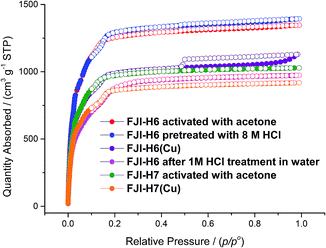 |
| Fig. 2 Experimental N2 adsorption isotherms for FJI-H6, FJI-H6(Cu), FJI-H7 and FJI-H7(Cu). | |
Since the Zr6O4(OH)4 and Hf6O4(OH)4 clusters are both highly connected with twelve carboxylate groups, FJI-H6 and FJI-H7 are expected to have a high stability. To test their stabilities, we immersed a microcrystalline sample of FJI-H6 or FJI-H7 into water with various pH values for 24 h (see Fig. S2†). The PXRD patterns of the resulting samples match well with the simulated ones, which suggests that FJI-H6 and FJI-H7 retain their crystallinity. In particular, FJI-H6 has high stability in acid. As seen in Fig. 2, the curves and the adsorption values for the sample treated with 8 M HCl do not deviate much from those of the untreated sample.
Incorporating Cu2+ ions into the open porphyrin rings via SCSC transformations
Considering that in both FJI-H6 and FJI-H7 two nitrogen atoms of the porphyrin ring are not deprotonated, we attempted to introduce a second kind of metal ion into the framework. Immersing single-crystals of FJI-H6 or FJI-H7 into a solution of 0.5 M Cu(NO3)2 in N,N-dimethyl formamide (DMF) at 85 °C for 72 h results in metallated FJI-H6(Cu) or FJI-H7(Cu). As anticipated, single crystal X-ray structural analysis definitively shows that in both FJI-H6 and FJI-H7 the Cu2+ ions have been embedded in the porphyrin rings. The Cu2+ ion is in a square planar N4 coordination environment with two axial sites exposed, which is typical for divalent metal ions in metal–porphyrin complexes.19 We believe that, although there are several examples of exchanging metal ions in porphyrin MOFs, this is the first observation of incorporating metal ions into open porphyrin rings via SCSC transformations in porphyrin MOFs. N2 adsorption measurements at 77 K for FJI-H6(Cu) also show a type I isotherm. At 1 atm, FJI-H6(Cu) has the maximum N2 adsorption of 1128 cm3 g−1, which is smaller than that of FJI-H6. Accordingly, the BET surface area of FJI-H6(Cu) is reduced to 3731 m2 g−1. Similarly, the maximum N2 adsorption and BET surface area of FJI-H7(Cu) (918 cm3 g−1 and 3195 m2 g−1, respectively) are also lower than those of FJI-H7. The reason may be ascribed to the introduction of Cu2+ ions, which can slightly diminish the surface area.
Cycloaddition reactions of CO2 with epoxides
Recently, owing to global warming, efficient CO2 capture and storage is urgently needed to reduce CO2 emissions before scientists find a practical clean energy. If we can convert this abundant inorganic waste into usable organic chemicals utilizing reasonable reactions at ambient conditions, the above problem can be perfectly solved. One practical method is the synthesis of cyclic carbonates from CO2 and epoxides, which have extensive applications as degreasers, polar aprotic solvents and electrolytes in lithium ion batteries. Though many catalysts have been explored for the above reaction, metalloporphyrins show relatively high catalytic activity.20 Hence, we have evaluated FJI-H6, FJI-H6(Cu), FJI-H7 and FJI-H7(Cu) as heterogeneous catalysts for the cycloaddition reaction of CO2 with epoxides (Scheme 1). Typically, 25.5 mmol 3-chloropropylene oxide, 0.51 mmol (2.0 mol%) tetrabutylammonium bromide and 0.051 mmol (0.2 mol%) catalyst were added to a thick-walled glass tube with a stirring bar. The tube was placed under vacuum and then purged with CO2. The above cycle was repeated three times. Finally, the pressure of CO2 was set as 1 atm. The mixture was stirred at 25 °C for 60 hours. Analysis of the resulting solution by gas chromatography indicated that 52.6%, 61.8%, 64% and 66.5% of the epoxide was converted into the cyclic carbonate for FJI-H6, FJI-H6(Cu), FJI-H7 and FJI-H7(Cu), respectively. Though the yields are not very high compared with homogeneous catalysts, it is nevertheless promising considering the low temperature and pressure. Compared with FJI-H6 or FJI-H7, FJI-H6(Cu) or FJI-H7(Cu) has a higher catalytic ability. The reason may be that, as a Lewis catalytic site, the embedded Cu(II) ion in the porphyrin ring contributes to some extent. At the same time, the Hf-based MOFs FJI-H7 and FJI-H7(Cu) have higher catalytic abilities than the corresponding Zr-based MOFs FJI-H6 and FJI-H6(Cu), respectively since the Hf ion is more oxophilic than the Zr ion and acts as a stronger Lewis acid. Additionally, the PXRD patterns of FJI-H6(Cu), FJI-H7 and FJI-H7(Cu) after catalyses are in good agreement with the simulated ones (see Fig. S2†), which further demonstrates that they all retain their framework. However, it is a pity that FJI-H6 lost its crystallinity during the catalytic process.
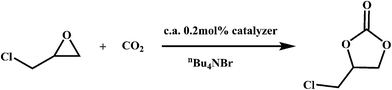 |
| Scheme 1 Cycloaddition reactions of CO2 with epoxide catalyzed by FJI-H6(Cu), FJI-H7 and FJI-H7(Cu). | |
Conclusions
In conclusion, we report the design and synthesis of two ultra-stable MOFs FJI-H6 and FJI-H7, which both feature 2.5 nm cages. In particular, FJI-H6 has a high BET surface area of 5033 m2 g−1. Due to the high connectivity of the M6O4(OH)4 clusters (M = Zr and Hf), FJI-H6 and FJI-H7 are stable in water with pH values ranging from 0 to 10. Interestingly, they can undergo a single-crystal to single-crystal transformation to embed Cu2+ ions into the porphyrin rings, which also indicates their high chemical stability. Additionally, preliminary catalysis evaluation shows that FJI-H6(Cu), FJI-H7 and FJI-H7(Cu) exhibit promising catalytic capacity for converting CO2 and epoxides into cyclic carbonates at low temperature and pressure. Consequently, FJI-H6, FJI-H7 and their derivatives may be applied in catalysis due to their high surface area, ultra-high stability and easy post-modification. Further research is ongoing.
Acknowledgements
We thank National Nature Science Foundation of China for the financial support (21131006, 21390392 and 21371169).
Notes and references
-
(a) J. Liu, L. Chen, H. Cui, J. Zhang, L. Zhang and C. Y. Su, Chem. Soc. Rev., 2014, 43, 6011–6061 RSC;
(b) A. Dhakshinamoorthy and H. Garcia, Chem. Soc. Rev., 2014, 43, 5750–5765 RSC;
(c) M. Yoon, R. Srirambalaji and K. Kim, Chem. Rev., 2012, 112, 1196–1231 CrossRef CAS PubMed;
(d) T. Zhang and W. B. Lin, Chem. Soc. Rev., 2014, 43, 5982–5993 RSC.
-
(a) J. R. Li, J. Sculley and H. C. Zhou, Chem. Rev., 2012, 112, 869–932 CrossRef CAS PubMed;
(b) M. P. Suh, H. J. Park, T. K. Prasad and D. W. Lim, Chem. Rev., 2012, 112, 782–835 CrossRef CAS PubMed;
(c) K. Sumida, D. L. Rogow, J. A. Mason, T. M. McDonald, E. D. Bloch, Z. R. Herm, T. H. Bae and J. R. Long, Chem. Rev., 2012, 112, 724–781 CrossRef CAS PubMed;
(d) Y. He, W. Zhou, G. Qian and B. Chen, Chem. Soc. Rev., 2014, 43, 5657–5678 RSC;
(e) S. L. Qiu, M. Xue and G. S. Zhu, Chem. Soc. Rev., 2014, 43, 6116–6140 RSC;
(f) R. B. Getman, Y. S. Bae, C. E. Wilmer and R. Q. Snurr, Chem. Rev., 2012, 112, 703–723 CrossRef CAS PubMed.
- P. Horcajada, R. Gref, T. Baati, P. K. Allan, G. Maurin, P. Couvreur, G. Ferey, R. E. Morris and C. Serre, Chem. Rev., 2012, 112, 1232–1268 CrossRef CAS PubMed.
-
(a) T. Devic and C. Serre, Chem. Soc. Rev., 2014, 43, 6097–6115 RSC;
(b) D. W. Feng, Z. Y. Gu, J. R. Li, H. L. Jiang, Z. W. Wei and H. C. Zhou, Angew. Chem., Int. Ed., 2012, 51, 10307–10310 CrossRef CAS PubMed;
(c) M. Zhang, Y. P. Chen, M. Bosch, T. Gentle 3rd, K. Wang, D. Feng, Z. U. Wang and H. C. Zhou, Angew. Chem., Int. Ed., 2014, 53, 815–818 CrossRef CAS PubMed;
(d) H. L. Jiang, D. Feng, K. Wang, Z. Y. Gu, Z. Wei, Y. P. Chen and H. C. Zhou, J. Am. Chem. Soc., 2013, 135, 13934–13938 CrossRef CAS PubMed;
(e) D. Feng, W. C. Chung, Z. Wei, Z. Y. Gu, H. L. Jiang, Y. P. Chen, D. J. Darensbourg and H. C. Zhou, J. Am. Chem. Soc., 2013, 135, 17105–17110 CrossRef CAS PubMed.
-
(a) H. Furukawa, N. Ko, Y. B. Go, N. Aratani, S. B. Choi, E. Choi, A. O. Yazaydin, R. Q. Snurr, M. O'Keeffe, J. Kim and O. M. Yaghi, Science, 2010, 329, 424–428 CrossRef CAS PubMed;
(b) B. B. Tu, Q. Q. Pang, D. F. Wu, Y. N. Song, L. H. Weng and Q. W. Li, J. Am. Chem. Soc., 2014, 136, 14465–14471 CrossRef CAS PubMed.
-
(a) L. Li, S. F. Tang, C. Wang, X. X. Lv, M. Jiang, H. Z. Wu and X. B. Zhao, Chem. Commun., 2014, 50, 2304–2307 RSC;
(b) A. Schaate, P. Roy, T. Preusse, S. J. Lohmeier, A. Godt and P. Behrens, Chem.–Eur. J., 2011, 17, 9320–9325 CrossRef CAS PubMed;
(c) J. H. Cavka, S. Jakobsen, U. Olsbye, N. Guillou, C. Lamberti, S. Bordiga and K. P. Lillerud, J. Am. Chem. Soc., 2008, 130, 13850–13851 CrossRef PubMed;
(d) M. J. Katz, Z. J. Brown, Y. J. Colon, P. W. Siu, K. A. Scheidt, R. Q. Snurr, J. T. Hupp and O. K. Farha, Chem. Commun., 2013, 49, 9449–9451 RSC;
(e) S. Biswas and P. Van Der Voort, Eur. J. Inorg. Chem., 2013, 2154–2160 CrossRef CAS PubMed;
(f) V. Bon, I. Senkovska, I. A. Baburin and S. Kaskel, Cryst. Growth Des., 2013, 13, 1231–1237 CrossRef CAS.
-
(a) H. Wu, T. Yildirim and W. Zhou, J. Phys. Chem. Lett., 2013, 4, 925–930 CrossRef CAS;
(b) H. Furukawa, F. Gandara, Y. B. Zhang, J. Jiang, W. L. Queen, M. R. Hudson and O. M. Yaghi, J. Am. Chem. Soc., 2014, 136, 4369–4381 CrossRef CAS PubMed.
- H. Wu, Y. S. Chua, V. Krungleviciute, M. Tyagi, P. Chen, T. Yildirim and W. Zhou, J. Am. Chem. Soc., 2013, 135, 10525–10532 CrossRef CAS PubMed.
-
(a) D. Sun, Y. Fu, W. Liu, L. Ye, D. Wang, L. Yang, X. Fu and Z. Li, Chem.–Eur. J., 2013, 19, 14279–14285 CrossRef CAS PubMed;
(b) W. Morris, B. Volosskiy, S. Demir, F. Gandara, P. L. McGrier, H. Furukawa, D. Cascio, J. F. Stoddart and O. M. Yaghi, Inorg. Chem., 2012, 51, 6443–6445 CrossRef CAS PubMed;
(c) P. Xydias, I. Spanopoulos, E. Klontzas, G. E. Froudakis and P. N. Trikalitis, Inorg. Chem., 2014, 53, 679–681 CrossRef CAS PubMed;
(d) J. M. Falkowski, T. Sawano, T. Zhang, G. Tsun, Y. Chen, J. V. Lockard and W. Lin, J. Am. Chem. Soc., 2014, 136, 5213–5216 CrossRef CAS PubMed;
(e) H. Fei, J. Shin, Y. S. Meng, M. Adelhardt, J. Sutter, K. Meyer and S. M. Cohen, J. Am. Chem. Soc., 2014, 136, 4965–4973 CrossRef CAS PubMed;
(f) K. Manna, T. Zhang and W. B. Lin, J. Am. Chem. Soc., 2014, 136, 6566–6569 CrossRef CAS PubMed;
(g) C. Wang, K. E. deKrafft and W. B. Lin, J. Am. Chem. Soc., 2012, 134, 7211–7214 CrossRef CAS PubMed;
(h) C. Wang, J. L. Wang and W. B. Lin, J. Am. Chem. Soc., 2012, 134, 19895–19908 CrossRef CAS PubMed;
(i) C. Wang, Z. Xie, K. E. deKrafft and W. Lin, J. Am. Chem. Soc., 2011, 133, 13445–13454 CrossRef CAS PubMed;
(j) K. K. Yee, N. Reimer, J. Liu, S. Y. Cheng, S. M. Yiu, J. Weber, N. Stock and Z. Xu, J. Am. Chem. Soc., 2013, 135, 7795–7798 CrossRef CAS PubMed.
-
(a) O. V. Gutov, W. Bury, D. A. Gomez-Gualdron, V. Krungleviciute, D. Fairen-Jimenez, J. E. Mondloch, A. A. Sarjeant, S. S. Al-Juaid, R. Q. Snurr, J. T. Hupp, T. Yildirim and O. K. Farha, Chem.–Eur. J., 2014, 20, 12389–12393 CrossRef CAS PubMed;
(b) Z. Wei, Z. Y. Gu, R. K. Arvapally, Y. P. Chen, R. N. McDougald Jr, J. F. Ivy, A. A. Yakovenko, D. Feng, M. A. Omary and H. C. Zhou, J. Am. Chem. Soc., 2014, 136, 8269–8276 CrossRef CAS PubMed;
(c) D. Feng, Z. Y. Gu, Y. P. Chen, J. Park, Z. Wei, Y. Sun, M. Bosch, S. Yuan and H. C. Zhou, J. Am. Chem. Soc., 2014, 136, 17714–17717 CrossRef CAS PubMed;
(d) S. B. Kalidindi, S. Nayak, M. E. Briggs, S. Jansat, A. P. Katsoulidis, G. J. Miller, J. E. Warren, D. Antypov, F. Cora, B. Slater, M. R. Prestly, C. Marti-Gastaldo and M. J. Rosseinsky, Angew. Chem., Int. Ed., 2015, 54, 221–226 CrossRef CAS PubMed;
(e) T. C. Wang, W. Bury, D. A. Gomez-Gualdron, N. A. Vermeulen, J. E. Mondloch, P. Deria, K. Zhang, P. Z. Moghadam, A. A. Sarjeant, R. Q. Snurr, J. F. Stoddart, J. T. Hupp and O. K. Farha, J. Am. Chem. Soc., 2015, 137, 3585–3591 CrossRef CAS PubMed;
(f) Q. Lin, X. Bu, A. Kong, C. Mao, X. Zhao, F. Bu and P. Feng, J. Am. Chem. Soc., 2015, 137, 2235–2238 CrossRef CAS PubMed.
-
(a) V. Bon, V. Senkovskyy, I. Senkovska and S. Kaskel, Chem. Commun., 2012, 48, 8407–8409 RSC;
(b) D. Feng, H. L. Jiang, Y. P. Chen, Z. Y. Gu, Z. Wei and H. C. Zhou, Inorg. Chem., 2013, 52, 12661–12667 CrossRef CAS PubMed;
(c) M. H. Beyzavi, R. C. Klet, S. Tussupbayev, J. Borycz, N. A. Vermeulen, C. J. Cramer, J. F. Stoddart, J. T. Hupp and O. K. Farha, J. Am. Chem. Soc., 2014, 136, 15861–15864 CrossRef CAS PubMed.
- Just prior to the submission of this manuscript, a similar strategy was reported by H. C. Zhou and his coworkers J. Am. Chem. Soc., 2015, 137, 413, whose work focuses on tuning the porosity of Zr-based MOFs through ligand design and variation. During the submission and revision of this manuscript, X. H. Bu and his coworkers discussed the ligand and heteroatom effect for optimizing ORR catalysts J. Am. Chem. Soc., 2015, 137, 2235; R. Q. Snurr and his coworkers synthesized a series of Zr-based MOFs employing a linker expansion approach and provided insight into the applicability of BET theory J. Am. Chem. Soc., 2015, 137, 3585. However, our work focuses not only on the syntheses of Hf and Zr-based MOFs, but also on the SCSC transformations and catalyses of the Zr and Hf-based MOFs.
- T. F. Liu, D. Feng, Y. P. Chen, L. Zou, M. Bosch, S. Yuan, Z. Wei, S. Fordham, K. Wang and H. C. Zhou, J. Am. Chem. Soc., 2015, 137, 413–419 CrossRef CAS PubMed.
- A. L. Spek, J. Appl. Crystallogr., 2003, 36, 7 CrossRef CAS.
- P. Deria, J. E. Mondloch, E. Tylianakis, P. Ghosh, W. Bury, R. Q. Snurr, J. T. Hupp and O. K. Farha, J. Am. Chem. Soc., 2013, 135, 16801–16804 CrossRef CAS PubMed.
- H. Sarkisov and A. Harrison, Mol. Simul., 2011, 37, 1248–1257 CrossRef.
- S. Q. Ma and H. C. Zhou, J. Am. Chem. Soc., 2006, 128, 11734–11735 CrossRef CAS PubMed.
- Y. Yan, M. Suyetin, E. Bichoutskaia, A. J. Blake, D. R. Allan, S. A. Barnett and M. Schroder, Chem. Sci., 2013, 4, 1731–1736 RSC.
-
(a) W. Y. Gao, M. Chrzanowski and S. Q. Ma, Chem. Soc. Rev., 2014, 43, 5841–5866 RSC;
(b) X. S. Wang, M. Chrzanowski, L. Wojtas, Y. S. Chen and S. Ma, Chem.–Eur. J., 2013, 19, 3297–3301 CrossRef CAS PubMed;
(c) Q. Z. Zha, X. Rui, T. T. Wei and Y. S. Xie, CrystEngComm, 2014, 16, 7371–7384 RSC;
(d) Z. J. Zhang, L. Wojtas, M. Eddaoudi and M. J. Zaworotko, J. Am. Chem. Soc., 2013, 135, 5982–5985 CrossRef CAS PubMed.
- T. Ema, Y. Miyazaki, J. Shimonishi, C. Maeda and J. Y. Hasegawa, J. Am. Chem. Soc., 2014, 136, 15270–15279 CrossRef CAS PubMed.
Footnote |
† Electronic supplementary information (ESI) available: General experimental, syntheses and characterization of the complexes mentioned in the manuscript, details of the single crystal diffraction experiments, PXRD, TG and additional figures. CCDC 1043280, 1043281, 1043914 and 1043915. For ESI and crystallographic data in CIF or other electronic format see DOI: 10.1039/c5sc00213c |
|
This journal is © The Royal Society of Chemistry 2015 |