DOI:
10.1039/C5RA12654A
(Paper)
RSC Adv., 2015,
5, 63080-63086
Synthesis, structure, physical properties and OLED application of pyrazine–triphenylamine fused conjugated compounds†
Received
30th June 2015
, Accepted 13th July 2015
First published on 13th July 2015
Abstract
In this paper, several donor–acceptor compounds using pyrazine and triphenylamine as building units have been synthesized and fully characterized. The physical properties and theoretical calculations were also investigated. In addition, the OLED devices using these compounds as active elements have been fabricated and all devices display very good performance. Specifically, the maximum EQE for 2 is 7.37%, which is higher than the theoretical limit for fluorescent emitters (5%), indicating that the mechanism for compound 2 is different from traditional fluorescent dyes. Our results suggest that D–A molecules could be a good approach to improve the performance of OLED devices.
1 Introduction
Organic light emitting diodes (OLEDs) have potential applications in the field of flat-panel display due to their low weight, low power consumption, self-emitting properties, high luminous efficiency, wide viewing angle and high contrast.1 OLEDs normally have three layers including a hole-transporting layer, a light-emitting layer and an electron-transporting layer, which are sandwiched together between two electrodes. The ideal compounds to approach better performance of OLEDs are dipolar D–A molecules, which incorporate both hole-transport units and electron-transport segments. There are two reasons for such design: (1) the hole mobility in the D–A molecules is slightly lower than that in a single hole-transporting layer, thus facilitating the exciton formation inside the layer; and (2) the low-lying LUMO of D–A molecules is helpful to the capture of electrons released from the cathode, which would improve the performance.2 In fact, many emissive compounds incorporating anthracene,3 pyrene,4 fluorine5 and triphenylamine6 segments have been synthesized and all compounds displays good performance in OLEDs. It is worthy to note that molecular geometry has two-faced effects on the electroluminescent (EL) efficiency: on one hand, planar geometry is very important to help the delocalization of π-electrons and improve the EL efficiency; on the other hand, non-planar geometry can provide steric hindrance that reduces the molecular aggregation in the solid state, thus increasing the EL efficiency.7
As a strong electron donor, triphenylamine has a unique propeller-like structure, and its distorted geometry diminishes the intermolecular interactions and favors efficient fluorescence.8 Thus, triphenylamine has been considered as an excellent hole transport material in OLED devices and memory devices.6 On the other hand, pyrazine units have been widely demonstrated as the electron acceptor to construct several electron-deficient structures.9 Moreover, pyrazine units can be easy to be functionalized by donor units, acceptor substituents, extended π-conjugation species, or bulky groups to form new conjugated materials.10 In this work, we are more interested in preparing a series of pyrazine–triphenylamine fused conjugated compounds for applications in OLEDs because (1) the LUMO and bandgap of these D–A molecules could be tuned through choosing the different electron-withdrawing abilities of the acceptor groups; (2) the fluorescence efficiency could also be modified by optimizing the intramolecular charge-transfer (ICT) strength; (3) the introduction of fused aromatic rings into the push–pull system could also lead to an enhanced carrier mobility and a lower band gap.11 Here, we want to investigate how different groups such as strong electron-acceptor cyano groups, moderate electron-acceptor pyridine groups and bulky 9,9-bis(2-ethylhexyl)fluorine to affect the structures (molecular stacking), physical properties and their performance in OLEDs.
2 Experimental section
2.1 Materials
Most reagents were purchased from Alfa Aesar or Aldrich and used as received unless otherwise noted. All the solvents used in photophysical measurements and electrochemical measurements were of HPLC grade quality. All other solvents were obtained commercially and purified using standard procedures.
2.2 Characterization
Using CDCl3 as a solvent and tetramethylsilane (TMS) as the internal standard, 1H NMR and 13C NMR spectra were measured on a Bruker Advance 300 MHz NMR spectrometer at ambient temperature. UV/Vis absorption spectra were carried out at room temperature with a Shimadzu UV-3600 spectrophotometer. High resolution mass spectra (HRMS) were recorded on a Waters Q-Tof premier™ mass spectrometer. Cyclic voltammetry measurements were carried out on a CHI660 electrochemical analyzer. Glassy carbon (diameter: 1.6 mm; area 0.02 cm2) was used as a working electrode, platinum wires were used as counter electrode and reference electrode, respectively. Fc+/Fc was used as an internal standard. Potentials were recorded versus Fc+/Fc in a solution of anhydrous dichloromethane (DCM) with 0.1 M tetrabutylammonium hexafluorophosphate (TBAPF6) as a supporting electrolyte at a scan rate of 50 mV s−1.
2.3 Fabrication of the OLED devices
The device structure is ITO/MoO3 (2 nm)/NPB (50 nm)/CBP: X wt% dye (20 nm)/Bphen (50 nm)/LiF (1 nm)/Al (150 nm), where ITO is anode, MoO3 is hole injection layer, NPB is hole transporting layer, CBP: X wt% dye is the emission layer, Bphen is the electron transporting layer, LiF is the electron injection layer and Al is the cathode.12 The doping concentrations of dye in CBP are 100% (neat dye layer), 50%, 10% and 3%, respectively.
2.4 Synthesis
As shown in Scheme 1, the starting material 4,4′-bis(N,N-diphenylamino) benzyl was prepared from TPA and oxalyl chloride through Friedel–Crafts acylation.13 And compounds 1–3 were synthesized by condensing 4,4′-bis (N,N-diphenylamino)benzyl and different kinds of diamines and tetraamines including diaminomaleonitrile, 2,3-diaminopyridine and 2,3,6,7-tetraamino-9,9-bis(2-ethylhexyl)fluorene in acetic acid with a catalytic amount of IBX.14 Note that the 2,3,6,7-tetraamino-9,9-bis(2-ethylhexyl)fluorene cannot react with two equivalents of 4,4′-bis(N,N-diphenylamino)benzil to form two pyrazine units due to the steric hindrance. In fact, it only reacts with one equivalent of 4,4′-bis(N,N-diphenylamino)benzil and one equivalent of acetic acid (solvent) to form compound 3 containing a pyrazine unit and oxazole segment.15
 |
| Scheme 1 Synthesis route for compounds 1–3. Conditions: anhydrous acetic acid, Ar2, 2-iodoxybenzoic acid, 120 °C, 24 h. | |
Synthesis of compound 1: 4,4′-bis(N,N-diphenylamino)benzil (540 mg, 1.0 mmol) and diaminomaleonitrile (108 mg, 1.0 mmol) were dissolved in anhydrous acetic acid (50 mL) with catalytic amount of 2-iodoxybenzoic acid (IBX). The solution was refluxed for 24 hours under argon atmosphere. After that the crude product was concentrated in vacuo and purified by column chromatography (CH2Cl2/petroleum ether = 1/2), affording compounds 1 (542 mg, yield 88%).
1H NMR (300 MHz, CDCl3) δ 7.50 (d, 4H), 7.34–7.29 (m, 8H), 7.16–7.12 (m, 12H), 6.96–6.93 (d, 4H). 13C NMR (75 MHz, CDCl3) δ 154.09, 150.78, 146.55, 130.87, 129.76, 128.33, 127.54, 126.09, 124.80, 120.32, 113.77. HRMS (M + 1) = 617.2440 (calc. 617.2454).
Synthesis of compound 2: The procedure was the same with above except that diaminomaleonitrile was replaced by 2,3-diaminopyridine (109 mg, 1.0 mmol). This reaction gave compounds 2 (549 mg, yield 89%).
1H NMR (300 MHz, CDCl3) δ 9.10–9.09 (m, 1H), 8.47–8.44 (m, 1H), 7.67–7.64 (m, 1H), 7.59 (d, 2H), 7.49 (d, 2H), 7.31–7.27 (m, 7H), 7.15–7.13 (m, 8H), 7.10–7.08 (m, 3H)), 7.07–7.06 (m, 2H)), 7.04–7.00 (m, 3H). 13C NMR (75 MHz, CDCl3) δ 156.15, 154.56, 153.25, 149.73, 149.39, 149.18, 147.27, 147.24, 138.16, 136.00, 131.85, 131.45, 131.24, 130.86, 129.56, 125.45, 125.32, 124.76, 123.91, 123.85, 121.99, 121.41. HRMS (M + 1) = 618.2673 (calc. 618.2658).
Synthesis of compound 3: The procedure was the same with above except that 2,3-diaminopyridine was replaced by 2,3,6,7-tetraamino-9,9-bis(2-ethylhexyl)fluorene (596 mg, 1.0 mmol). This reaction gave compounds 3 (787 mg, yield 80%).
1H NMR (300 MHz, CDCl3) δ 8.45 (s, 1H), 8.13 (s, 1H), 8.09 (s, 1H), 7.72–7.69 (m, 1H), 7.54–7.52 (m, 1H), 7.51–7.44 (m, 5H), 7.29 (d, 6H), 7.14 (d, 8H), 7.09–7.04 (m, 8H). 13C NMR (75 MHz, CDCl3) δ 167.90, 164.67, 152.15, 149.17, 148.58, 147.46, 143.96, 141.77, 136.57, 132.62, 131.02, 129.50, 128.95, 125.11, 123.59, 122.32, 117.76, 111.66, 106.33, 68.31, 54.93, 46.33, 38.89, 34.92, 34.87, 33.66, 33.32, 32.07, 30.52, 29.84, 29.59, 29.51, 29.40, 29.24, 29.08, 28.05, 26.95, 26.67, 26.62, 24.90, 23.91, 23.13, 22.84, 22.77, 14.87, 14.26, 14.19, 13.98, 13.95, 11.11, 10.26. HRMS (M + 1) = 984.5613 (calc. 984.5508).
3 Results and discussion
3.1 Crystal structures
The structures and molecular packing of compounds 1–3 are shown in Fig. 1 (CCDC number for compounds 1–3 are 1401901, 1401902, and 1401903, respectively. For compound 1, the length of C–N bond in cyano group is 1.13–1.14 Å, which is a typical C–N triple bond. The length of C–C bond connecting cyano group to pyrazine ring (1.45 Å) is between the average bond length of C–C single bond (1.54 Å) and aromatic C–C bond (double bond 1.33 Å and aromatic 1.40 Å). For compounds 2 and 3, the length of C–C bond connecting pyrazine ring to triphenylamine (1.48 Å) are also between the average bond length of C–C single bond and aromatic C–C bond while the length of C–N bond in triphenylamine unit (1.41–1.43 Å) is close to the average aromatic C–N bond length (C–N single bond 1.47 Å; double bond 1.34 Å; aromatic 1.43 Å). These phenomena indicate that strong donor–acceptor interactions exist in these molecules. The lengths of C–N bond of pentagon in compound 3 are 1.281 and 1.400 Å, separately. Note that compound 2 forms dimer via intermolecular N–H hydrogen bond. The adjacent molecules in compound 1 adopt head-to-tail arrangement to reduce the electron coupling effect due to the large molecule dipole moment.
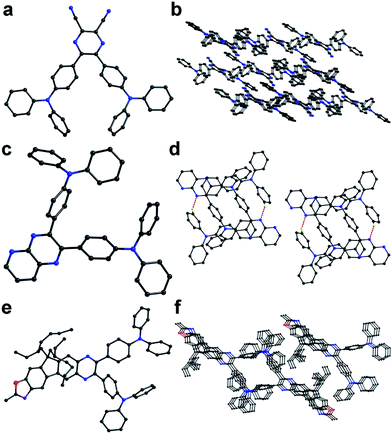 |
| Fig. 1 The crystal structures of compounds 1–3 (a, c and e) and the crystal packing diagrams of compounds 1–3 (b, d and f). | |
Table 1 The UV-Vis and fluorescence data of compounds 1–3
|
Abs λmax (nm) |
ε (M−1 cm−1) |
Fluor λmax (nm) |
фf1 |
1 |
468 |
39 540 |
593 |
0.02 |
2 |
430 |
23 540 |
568 |
0.09 |
3 |
428 |
46 900 |
528 |
0.99 |
Table 2 The CV data of compounds 1–3
|
Eox1/V |
Eox2/V |
Ered1/V |
Ered2/V |
HOMO |
LUMO |
Eg (eV) |
1 |
0.52 |
1.50 |
−0.29 |
−1.62 |
−5.32 |
−2.17 |
3.15 |
2 |
0.53 |
1.01 |
−1.74 |
— |
−5.33 |
−1.92 |
3.41 |
3 |
0.35 |
0.89 |
— |
— |
−5.15 |
−1.85 |
3.30 |
Table 3 The calculated data of compounds 1–3
|
HOMO (eV) |
LUMO (eV) |
Gap (eV) |
Dipole moment (D) |
1 |
−5.25 |
−2.49 |
2.76 |
11.92 |
2 |
−4.95 |
−2.04 |
2.91 |
1.0259 |
3 |
−4.85 |
−1.86 |
2.99 |
0.9586 |
Table 4 OLED device characteristics of compounds 1–3a
|
X (wt%) |
Von (V) |
Lmax (cd m−2) |
Max efficiency |
Efficiency@100 cd m−2 |
Efficiency@1000 cd m−2 |
CIE (x, y) |
EQE (%) |
CE (cd A−1) |
PE (lm/W) |
EQE (%) |
CE (cd A−1) |
PE (lm/W) |
EQE (%) |
CE (cd A−1) |
PE (lm/W) |
Von is turn-on voltage (the voltage when luminance reaches 1 cd m−2); Lmax is maximum luminance; EQE is external quantum efficiency; CE is current efficiency; PE is power efficiency. |
1 |
100 |
3.6 |
3270 |
0.89 |
1.48 |
1.23 |
0.57 |
1.03 |
0.63 |
0.22 |
0.60 |
0.36 |
(0.59, 0.40) |
50 |
3.4 |
4775 |
1.81 |
3.64 |
3.39 |
0.87 |
1.75 |
1.02 |
0.48 |
0.97 |
0.40 |
(0.56, 0.43) |
10 |
3.3 |
8202 |
3.12 |
9.23 |
8.05 |
1.31 |
2.20 |
3.93 |
0.78 |
2.31 |
0.93 |
(0.49, 0.50) |
3 |
3.3 |
11 550 |
4.01 |
16.12 |
15.35 |
1.30 |
4.57 |
2.51 |
0.84 |
2.95 |
1.14 |
(0.40, 0.57) |
2 |
100 |
3.0 |
7916 |
0.60 |
2.04 |
1.10 |
0.50 |
1.69 |
1.09 |
0.60 |
2.03 |
0.96 |
(0.41, 0.56) |
50 |
2.7 |
10 520 |
1.24 |
4.43 |
4.13 |
1.23 |
4.41 |
3.30 |
1.18 |
4.23 |
2.21 |
(0.39, 0.58) |
10 |
3.0 |
12 650 |
7.37 |
26.65 |
27.90 |
2.60 |
9.41 |
6.16 |
1.24 |
4.50 |
1.90 |
(0.33, 0.61) |
3 |
3.3 |
12 860 |
7.16 |
24.66 |
23.47 |
2.10 |
7.20 |
4.01 |
1.22 |
4.15 |
1.70 |
(0.29, 0.61) |
3 |
100 |
3.0 |
2378 |
1.36 |
3.93 |
3.51 |
1.22 |
3.51 |
2.40 |
0.61 |
1.78 |
0.75 |
(0.24, 0.55) |
50 |
2.9 |
11 860 |
2.04 |
6.24 |
5.64 |
2.02 |
6.18 |
4.60 |
1.64 |
5.02 |
2.51 |
(0.22, 0.58) |
10 |
3.3 |
11 120 |
2.89 |
7.98 |
8.35 |
2.05 |
5.68 |
3.50 |
1.42 |
3.93 |
1.64 |
(0.19, 0.51) |
3 |
3.6 |
10 200 |
2.09 |
5.13 |
4.13 |
1.81 |
4.45 |
2.63 |
1.33 |
3.27 |
1.37 |
(0.17, 0.44) |
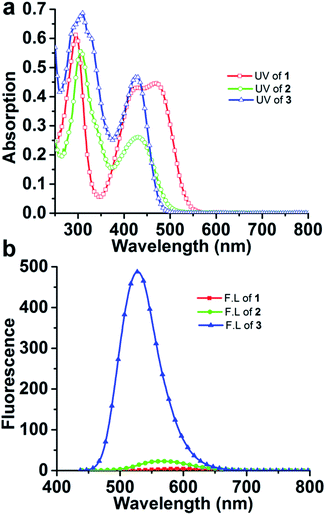 |
| Fig. 2 The UV-Vis (a) and fluorescence (b) spectra of compounds 1–3. They are measured in dichloromethane with the concentration of 10−5 M and 10−6 M, respectively. | |
3.2 Optical and electrochemical properties
The maximum absorption wavelengths of compounds 1–3 show a trend of 3 < 2<1, reflecting the substituent nature (Fig. 2 and Table 1). Fluorene is a weak electron donor, while pyridine is a moderate electron acceptor and cyano group is a strong electron acceptor. With the enhancement of the role of the electron acceptor, the efficiency of intramolecular charge-transfer (ICT) is improved, resulting a bathochromic absorption shift. This trend is also manifested in the emission characteristics. The emission intensity decreases significantly with a concomitant red shift in the emission wavelength from compound 3 to 1, which suggests that dipolar interactions have a great contribution to the non-radiative decay in these compounds.16 The lifetime of films of 1, 2 and 3 are measured by a streak camera system (Table S2†). The experimental details are described in (ref. 17). We can see that the PL decay rate increases from 1, 2 to 3, and the lifetime (the time at which the initial PL intensity (I0) decreases to the (I0/e)) are extracted to be 4.25, 3.27 and 2.12 ns for 1, 2 and 3, respectively.
As shown in Fig. 3, for compound 1, one reversible reduction wave and one irreversible reduction wave were investigated, while for compound 2, only one reversible reduction wave was found. Because the electron affiliation of cyano group is stronger than that of pyridine, the reduction potentials of compounds 1 are higher than that of compound 2 (Table 2).
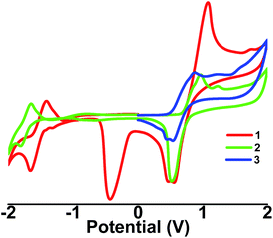 |
| Fig. 3 The CV spectra of compounds 1–3. | |
3.3 Theoretical calculation
The optimization for geometric structures of compounds 1–3 is carried out by Gaussian 09 program at the B3LYP/6-31G* level. The electronic distributions in the HOMO and LUMO for these compounds are shown in Fig. 4. The HOMOs of compounds 1 and 2 are delocalized over the whole molecule but the HOMO of compound 3 are delocalized over the triphenylamine groups. The LUMOs are delocalized on the cyano group, pyridine or quinoxaline. With the acceptor strength increasing in the order from compound 3 to compound 1, the donor–acceptor interactions are enhanced gradually, which induce a higher dipole moment and stabilize the HOMO and LUMO energy level. Since the LUMO is more sensitive than the HOMO in our case, the band gap becomes narrower (Table 3).
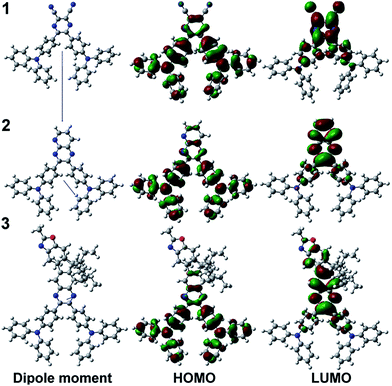 |
| Fig. 4 The dipole moment and molecular orbitals of compounds 1–3. | |
3.4 OLED performance
As shown in Fig. 5, the turn-on voltages for the devices range from 2.9 V to 3.6 V, which are within the normal range for OLEDs (Table 4). For doped emission layers, maximum brightness levels around or higher than 10
000 cd m−2 were achieved. All the dyes show good efficiencies (with EQE above 2%). Among them, compounds 1 and 2 show better performance. The maximum EQE for compounds 1 and 2 are 4.01% and 7.37%, respectively, both achieved at low doping concentration (less than 10 wt%). Especially for compound 2, the EQE is higher than the theoretical limit for fluorescent emitters (5%),18 indicating that the mechanism for compound 2 is different from traditional fluorescent dyes. The mechanism is still under investigation. The concentration dependence of device EQE for the dyes also shows some difference. For compounds 1 and 2, the concentration quenching effect is much stronger than compound 3. This can also inferred from the concentration dependent electroluminance (EL) spectrum. (Fig. S3-2†) For compounds 1and 2, their EL spectra shows larger redshift compared with that of compound 3. These results indicate that the introduction of bulk substituted groups can reduce the aggregation caused quenching (ACQ) effect by sacrificing the efficiency or raising the turn-on-voltage.19
 |
| Fig. 5 The current density–voltage–luminance curves (a, c and e) and external quantum efficiency curves (b, d and f) of compounds 1–3. | |
4 Conclusion
In summary, using one-step reaction between 1,2-diamine and 1,2-diketones, a series of D–A molecules containing triphenylamine segment and pyrazine unit have been synthesized and fully characterized. The modification of the pyrazine unit with different substituted groups such as strong electron-acceptor cyano groups, moderate electron-acceptor pyridine groups and large steric functional group 9,9-bis(2-ethylhexyl)fluorine gave different patterns of D–A compounds with different photoelectric properties. The OLED devices based on these compounds exhibit good efficiencies. In particular, CBP-doped devices using compounds 1 and 2 as active layers show maximum external quantum efficiency of 4.01% and 7.37%, respectively. These results indicate that these compounds have good applications in OLED devices.
Acknowledgements
Q. Z. acknowledges financial support from AcRF Tier 1 (RG 16/12 and RG 133/14) and Tier 2 (ARC 20/12 and ARC 2/13) from MOE, and the CREATE program (Nanomaterials for Energy and Water Management) from NRF.
References
-
(a) M. Gross, D. C. Muller, H.-G. Nothofer, U. Scherf, D. Neher, C. Brauchle and K. Meerholz, Nature, 2000, 405, 661 CrossRef CAS PubMed
;
(b) H. Li, A. S. Batsanov, K. C. Moss, H. L. Vaughan, F. B. Dias, K. T. Kamtekar, M. R. Bryce and A. P. Monkman, Chem. Commun., 2010, 46, 4812–4814 RSC
;
(c) H. Uoyama, K. Goushi, K. Shizu, H. Nomura and C. Adachi, Nature, 2012, 492, 234–238 CrossRef CAS PubMed
. - K. R. Justin Thomas, J. T. Lin, Y.-T. Tao and C.-H. Chuen, Chem. Mater., 2002, 14, 2796–2802 CrossRef
. -
(a) C.-J. Zheng, W.-M. Zhao, Z.-Q. Wang, D. Huang, J. Ye, X.-M. Ou, X.-H. Zhang, C.-S. Lee and S.-T. Lee, J. Mater. Chem., 2010, 20, 1560–1566 RSC
;
(b) P.-Y. Gu, Y. Zhao, J.-H. He, J. Zhang, C. Wang, Q.-F. Xu, J.-M. Lu, X. W. Sun and Q. Zhang, J. Org. Chem., 2015, 80, 3030–3035 CrossRef CAS PubMed
;
(c) J. Li, Y. Zhao, J. Lu, G. Li, J. Zhang, Y. Zhao, X. Sun and Q. Zhang, J. Org. Chem., 2015, 80, 109–113 CrossRef CAS PubMed
. -
(a) Z. Zhao, S. Chen, J. W. Y. Lam, P. Lu, Y. Zhong, K. S. Wong, H. S. Kwok and B. Z. Tang, Chem. Commun., 2010, 46, 2221–2223 RSC
;
(b) Q. Zhang, Y. Divayana, J. Xiao, Z. Wang, E. R. T. Tiekink, H. M. Doung, H. Zhang, F. Boey, X. W. Sun and F. Wudl, Chem.–Eur. J., 2010, 16, 7422 CrossRef CAS PubMed
;
(c) J. Xiao, Y. Divayana, Q. Zhang, H. M. Doung, H. Zhang, F. Boey, X. W. Sun and F. Wudl, J. Mater. Chem., 2010, 20, 8167–8170 RSC
;
(d) J. Xiao, B. Yang, J. I. Wong, Y. Liu, F. Wei, K. J. Tan, X. Teng, Y. Wu, L. Huang, C. Kloc, F. Boey, J. Ma, H. Zhang, H. Yang and Q. Zhang, Org. Lett., 2011, 13, 3004–3007 CrossRef CAS PubMed
;
(e) J. Xiao, S. Liu, Y. Liu, L. Ji, X. Liu, H. Zhang, X. Sun and Q. Zhang, Chem.–Asian J., 2012, 7, 561–564 CrossRef CAS PubMed
;
(f) G. Li, A. A. Putu, J. Gao, Y. Divayana, W. Chen, Y. Zhao, X. W. Sun and Q. Zhang, Asian J. Org. Chem., 2012, 1, 346–351 CrossRef CAS PubMed
. - F. Polo, F. Rizzo, M. Veiga-Gutierrez, L. De Cola and S. Quici, J. Am. Chem. Soc., 2012, 134, 15402–15409 CrossRef CAS PubMed
. -
(a) P. Cias, C. Slugovc and G. Gescheidt, J. Phys. Chem. A, 2011, 115, 14519–14525 CrossRef CAS PubMed
;
(b) C. Wang, M. Yamashita, B. Hu, Y. Zhou, J. Wang, J. Wu, F. Huo, P. S. Lee, N. Aratani, H. Yamada and Q. Zhang, Asian J. Org. Chem., 2015, 4, 646–651 CrossRef CAS PubMed
;
(c) C. Wang, J. Wang, P. Li, J. Gao, S. Y. Tan, W. Xiong, B. Hu, P. S. Lee, Y. Zhao and Q. Zhang, Chem.–Asian J., 2014, 9, 779–783 CrossRef CAS PubMed
. -
(a) Z. Guo, W. Zhu and H. Tian, Chem. Commun., 2012, 48, 6073–6084 RSC
;
(b) H. Park, J. Lee, I. Kang, H. Y. Chu, J.-I. Lee, S.-K. Kwon and Y.-H. Kim, J. Mater. Chem., 2012, 22, 2695–2700 RSC
. - W. Z. Yuan, Y. Gong, S. Chen, X. Y. Shen, J. W. Y. Lam, P. Lu, Y. Lu, Z. Wang, R. Hu, N. Xie, H. S. Kwok, Y. Zhang, J. Z. Sun and B. Z. Tang, Chem. Mater., 2012, 24, 1518–1528 CrossRef CAS
. -
(a) E. Zhou, J. Cong, S. Yamakawa, Q. Wei, M. Nakamura, K. Tajima, C. Yang and K. Hashimoto, Macromolecules, 2010, 43, 2873–2879 CrossRef CAS
;
(b) Y. Zhu, R. D. Champion and S. A. Jenekhe, Macromolecules, 2006, 39, 8712–8719 CrossRef CAS
. -
(a) Naraso, J.-i. Nishida, M. Tomura and Y. Yamashita, Synth. Met., 2005, 153, 389–392 CrossRef CAS PubMed
;
(b) L. Dokladalova, F. Bures, W. Kuznik, I. V. Kityk, A. Wojciechowski, T. Mikysek, N. Almonasy, M. Ramaiyan, Z. Padelkova, J. Kulhanek and M. Ludwig, Org. Biomol. Chem., 2014, 12, 5517–5527 RSC
;
(c) X. Zhang, J. Mao, D. Wang, X. Li, J. Yang, Z. Shen, W. Wu, J. Li, H. Ågren and J. Hua, ACS Appl. Mater. Interfaces, 2015, 7, 2760–2771 CrossRef CAS PubMed
;
(d) X. Lu, S. Fan, J. Wu, X. Jia, Z.-S. Wang and G. Zhou, J. Org. Chem., 2014, 79, 6480–6489 CrossRef CAS PubMed
;
(e) L. V. Brownell, K. A. Robins, Y. Jeong, Y. Lee and D.-C. Lee, J. Phys. Chem. C, 2013, 117, 25236–25247 CrossRef CAS
;
(f) R. L. Schwiderski and S. C. Rasmussen, J. Org. Chem., 2013, 78, 5453–5462 CrossRef CAS PubMed
;
(g) B. L. Watson, B. D. Sherman, A. L. Moore, T. A. Moore and D. Gust, Phys. Chem. Chem. Phys., 2015, 17, 15788–15796 RSC
;
(h) J. Ohshita, Y. Adachi, D. Tanaka, M. Nakashima and Y. Ooyama, RSC Adv., 2015, 5, 36673–36679 RSC
;
(i) S. Demir, M. Nippe, M. I. Gonzalez and J. R. Long, Chem. Sci., 2014, 5, 4701–4711 RSC
;
(j) Y. Ooyama, K. Uenaka, Y. Harima and J. Ohshita, RSC Adv., 2014, 4, 30225–30228 RSC
;
(k) H. Yokoi, N. Wachi, S. Hiroto and H. Shinokubo, Chem. Commun., 2014, 50, 2715–2717 RSC
. - S. J. Evenson, M. J. Mumm, K. I. Pokhodnya and S. C. Rasmussen, Macromolecules, 2011, 44, 835–841 CrossRef CAS
. -
(a) B. S. Kim and J. Y. Lee, Org. Electron., 2015, 21, 100–105 CrossRef CAS PubMed
;
(b) Q.-D. Ou, L. Zhou, Y.-Q. Li, J.-D. Chen, C. Li, S. Shen and J.-X. Tang, Adv. Opt. Mater., 2015, 3, 87–94 CrossRef CAS PubMed
. - C. Wang, B. Hu, J. Wang, J. Gao, G. Li, W.-W. Xiong, B. Zou, M. Suzuki, N. Aratani, H. Yamada, F. Huo, P. S. Lee and Q. Zhang, Chem.–Asian J., 2015, 10, 116–119 CrossRef PubMed
. - B. D. Lindner, J. U. Engelhart, O. Tverskoy, A. L. Appleton, F. Rominger, A. Peters, H.-J. Himmel and U. H. F. Bunz, Angew. Chem., Int. Ed., 2011, 50, 8588–8591 CrossRef CAS PubMed
. - J. F. Mike, A. J. Makowski and M. Jeffries-El, Org. Lett., 2008, 10, 4915–4918 CrossRef CAS PubMed
. - B. R. Cho, K. H. Son, S. H. Lee, Y.-S. Song, Y.-K. Lee, S.-J. Jeon, J. H. Choi, H. Lee and M. Cho, J. Am. Chem. Soc., 2001, 123, 10039–10045 CrossRef CAS PubMed
. -
(a) Y. Wang, V. D. Ta, Y. Gao, T. C. He, R. Chen, E. Mutlugun, H. V. Demir and H. D. Sun, Adv. Mater., 2014, 26, 2954–2961 CrossRef CAS PubMed
;
(b) Y. Wang, K. S. Leck, V. D. Ta, R. Chen, V. Nalla, Y. Gao, T. He, H. V. Demir and H. Sun, Adv. Mater., 2015, 27, 169–175 CrossRef CAS PubMed
. - T. Higuchi, H. Nakanotani and C. Adachi, Adv. Mater., 2015, 27, 2019–2023 CrossRef CAS PubMed
. - J. Luo, Y. Zhou, Z.-Q. Niu, Q.-F. Zhou, Y. Ma and J. Pei, J. Am. Chem. Soc., 2007, 129, 11314–11315 CrossRef CAS PubMed
.
Footnote |
† Electronic supplementary information (ESI) available. CCDC 1401901–1401903. For ESI and crystallographic data in CIF or other electronic format see DOI: 10.1039/c5ra12654a |
|
This journal is © The Royal Society of Chemistry 2015 |
Click here to see how this site uses Cookies. View our privacy policy here.