DOI:
10.1039/C5RA03805G
(Paper)
RSC Adv., 2015,
5, 32643-32656
Model peptides containing the 3-sulfanyl-norbornene amino acid, a conformationally constrained cysteine analogue effective inducer of 310-helix secondary structures†
Received
4th March 2015
, Accepted 27th March 2015
First published on 27th March 2015
Abstract
The properties of the constrained tetrasubstituted 3-sulfanylnorbornene amino acid (NRB), when inserted in Ala-Aib model peptides, were extensively studied. The conformational behaviour of these models was evaluated by theoretical calculations, spectroscopic analyses and by X-ray crystallography. Taken together, our data confirm that both (R,R,R,S)- and (S,S,S,R)-NRB enantiomers possess a strong helicogenic effect when inserted in short Ala-Aib sequences, suggesting that the rigid norbornane core has a positive effect on the ability to stabilize helical secondary structures. This information will be essential for future applications in the rational design of conformationally stable peptides targeted on protein–protein interaction (PPI) surfaces.
Introduction
Protein–protein interactions (PPI) represent a central event in biological pathways and the possibility to modulate such interactions is considered of great importance.1 Several efforts were devoted to discover new small drug-like molecules acting as modulators of PPI, but sometimes providing unsatisfactory results.2 Recent strategies are focused on the use of ad hoc designed natural peptides,3 which, however, often have a low stability, bad pharmacokinetics and an unstable conformation.4 The exploitation of non-natural amino acids (AAs) in peptide synthesis is very useful to overcome these problems. Peptidomimetic sequences5 are inherently resistant to proteases and peptidases and can fold into well-ordered secondary structures consisting of helices, turns and sheets.6
Such stabilized secondary structures might be crucial to enhance the activity and selectivity of interaction with a specific biological target. In particular, Cα-tetrasubstituted α-amino acids (CTAAs) were used to stabilize bioactive helical peptides.7
In our laboratory, synthetic procedures for the preparation of several constrained CTAAs6b,8–11 were developed. Some of these were successfully used for the preparation of short peptides, which fold into stable helical structures. Clearly, the ability of a new amino acid to induce a defined secondary structure must be proved by experimental data. Moreover, the stereochemistry of the new AA is of fundamental importance: depending on the enantiomer chosen, a different conformational stability of the resulting isomeric peptide could be expected.6b
In the last few years interest toward Rac1 protein, whose activation is mediated by Tiam1, has increased for its role in cancer and cardiovascular diseases.12 In a recent article we described the design and synthesis of a new class of small molecules acting as Rac1-Tiam1 PPI inhibitors.13 These compounds, even if of great interest, showed, as other inhibitors reported so far, a potency too low for a potential use in therapy (low μM range).14
Preliminary studies performed in our laboratory identified a peptide sequence containing hot and warm spots in the 13 residue long helix of Tiam1, CR3 (I1187-L1199); however, this portion cannot be used as a stand-alone peptide inhibitor, since it is predicted to be disordered.15 Taking into account the above considerations, the introduction into the peptide sequence of one or more CTAAs would result in a helical structured more stable peptide. According to recent studies of our group,16 we chose the 3-benzylsulfanyl amino ester 1 containing the norbornene amino acid (NRB) as a CTAA. First of all we planned the preparation of model pentapeptides Ac-Ala-NRB-Ala-Aib-Ala-NH2 of general formula 2 (Fig. 1) by using both enantiomers of NRB. The conformational behavior of these models and the ability of the two different enantiomers of NRB to induce a defined secondary structure was evaluated by theoretical calculation, spectroscopic analyses, and by X-ray crystallography. These information, besides being of importance for developing new peptidomimetic inhibitors of Rac1-Tiam1 PPI, might lay the ground for future applications in the rational design of other new bioactive peptidomimetics.
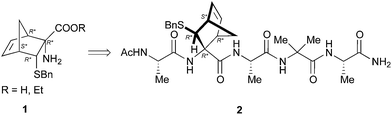 |
| Fig. 1 Pentapeptides from norbornene amino acid precursor. | |
Results and discussion
The synthesis of 1 (R = Et) previously described by us,10 was scaled up to 1.5 grams in order to assure the availability of a congruous amount of starting NRB.
For the preparation of pentapeptides 2 several strategies were tested.
Firstly, we planned to use the procedure depicted in Scheme 1, whose key step consists in coupling the diastereoisomeric dipeptides Ac-Ala-NRB-OH (3) with the TFA salt of the tripeptide H-Ala-Aib-Ala-NH2 (4), synthesized by solid phase synthesis.6b
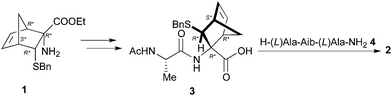 |
| Scheme 1 Original synthetic plan. | |
Accordingly, 1 was submitted to hydrolysis obtaining the fast conversion of the ester into acid in high yield but, as already observed in similar cases,10 together with the addition of HCl to the double bond. Operating in basic condition resulted in very slow reaction, low yield and purification difficulties. To overcome this problem the double bond of 1 was reduced providing 5 (Scheme 2), which was reacted with FmocAlaF as both reagent and resolving agent.17 Unfortunately, an inseparable mixture of the two expected diastereoisomers was obtained. Fmoc deprotection (piperidine, DMF) afforded the two diastereoisomers 6a and 6b (95% overall yield), which were partially separated by simple flash chromatography.
 |
| Scheme 2 Synthesis of pentapeptides: first synthetic approach. | |
The two compounds were analysed to ascertain their correct structure, but very little differences were observed in protons chemical shifts (1H NMR, see Experimental), the main difference being the methylenic protons of ester function (see ESI†). A confirmation of the correct structure and stereochemistry came from X-ray analyses. Suitable crystals were obtained only for one of the two diastereoisomers (6a: AcOEt
:
CHCl3 1
:
1; RT; 24 h). Finally, acetylation of 6a and 6b followed by hydrolysis of the ethyl esters provided 7a and 7b. Unfortunately, the hydrolysis led to racemization of the alanine stereocenter or to a partial cleavage of amides bond despite to numerous reaction conditions tested (NaOH, KOH, LiOH, or HCl 3 N, 6 N, 37%). However, we tried to couple the crude containing 7a with the tripeptide H-Ala-Aib-Ala-NH2 (4) operating in standard conditions (EDC 1.1 eq., HOAt 1.1 eq., DCM, 0 °C), but we obtained an inseparable mixture.
The main drawbacks of the synthetic way described above were the limited scalability of the known solid phase synthesis of the tripeptide H-Ala-Aib-Ala-NH2 (4)6b and the racemization events during the hydrolytic procedures. Consequently, first of all we set up a linear Boc chemistry solution strategy that allowed us to prepare the tripeptide 4 as a free amine in 50% overall yield on a gram scale (Scheme 3). The process consisted in the coupling between H-Ala-NH2 (9) or the dipeptide H-Aib-Ala-NH2 (11) with the Boc-protected AA 8 and, respectively, 12 in standard conditions (see Scheme 3). The coupling afforded respectively the dipeptide Boc-Aib-Ala-NH2 (10) and the tripeptide Boc-Ala-Aib-Ala-NH2 (13).
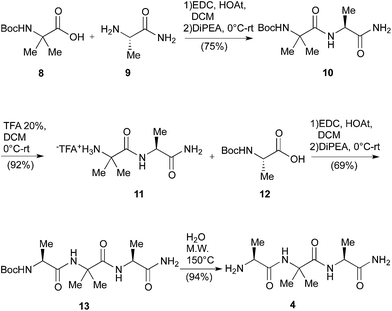 |
| Scheme 3 Gram scale synthesis of the tripeptide 4. | |
The Boc deprotection of 10 was, as usual, realized by treatment with TFA/DCM. On the other hand the deprotection of 13, affording the desired compound 4, was obtained by microwave (20 + 20 min, 150 °C) mediated thermal decomposition of Boc protection.18
In such way we avoided the formation of the corresponding TFA salt, negatively affecting the key coupling with the Boc protected NRB 15.
Having in hand a good protocol for the preparation of compound 4, we reconsidered the use of AA 1 and a different strategy was set up to obtain peptides 2.
First, the Boc-protected NRB 15 was prepared in good yield on gram scale according to standard protocols through the reaction of 1 with Boc anhydride, giving 14, followed by hydrolysis of the ester function in basic conditions (KOH 3 M in MeOH, Scheme 4). Compound 15 was coupled with the tripeptide 4 in the conditions reported in Scheme 4, affording the two expected diastereoisomeric tetrapeptides 16a and 16b (yield 75%) that were successfully separated by flash chromatography. The following microwave assisted Boc deprotection afforded compounds 17a (82%) and 17b (88%) without racemization.
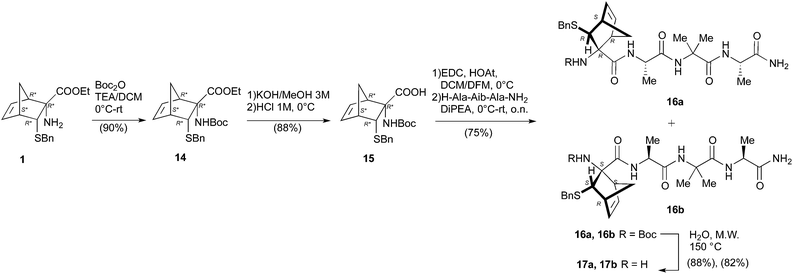 |
| Scheme 4 Synthesis of peptides 17a and 17b. | |
Peptides 17a and 17b were then reacted with Ac-Ala-OH in different conditions (Scheme 5). To optimize the yields and to minimize racemization, several synthetic protocols were tested which are reported, together with yields and percentage of racemization, in Table 1, starting from 17a (entries 1–5) and 17b (entries 6–10), respectively. The best conditions to obtain compound 2a from 17a resulted in using classic coupling condensation conditions (HOAt 1.1 eq., EDC 1.1 eq., DIPEA 2.2 eq., DCM, entry 1). In this way, pure compound 2a was obtained after HPLC purification (40%).
 |
| Scheme 5 Synthesis of projected peptide 2a and 2b. | |
Table 1 Reaction conditions for the synthesis of 2a and2b
Entry |
Reagent |
Solvent |
Reaction conditions |
T (°C) |
Yield (%) |
Racemization (%) |
1 |
17a |
DCM |
HOAt, EDC, DIPEA |
0–rt |
40 |
5 |
2 |
17a |
THF |
EEDQ, MW (70 Watt), air cooling, 30 min |
60–70 °C |
60 |
20 |
3 |
17a |
DCM |
HOBt, EDC, DIPEA |
0–rt |
5 |
— |
4 |
17a |
DCM |
HBTU, EDC |
0–rt |
— |
— |
5 |
17a |
DCM |
HBTU, DIPEA |
0–rt |
10 |
— |
6 |
17b |
THF |
EEDQ MW (70 Watt), air cooling, 30 min |
60–70 |
60 |
0 |
7 |
17b |
THF |
EEDQ |
0–rt |
35 |
0 |
8 |
17b |
DCM |
HOAt, EDC, DIPEA |
0–rt |
30 |
15 |
9 |
17b |
DCM |
HOAt, EDC, DIPEA |
0–rt |
— |
— |
10 |
17b |
DCM |
HBTU, DIPEA |
0–rt |
Complex mixture |
— |
In the case of 17b partially satisfactory results were obtained by using EEDQ (entry 7) as the condensing agent in THF as the solvent. Racemization process was avoided but the yield was still low.
It is well known that MWs are able to promote unfolding of peptides and this effect is exploited in solid phase peptide synthesizers, thus allowing a general increase of yields. Nevertheless, to our knowledge, few examples are present in the literature concerning the use of MW in solution peptide synthesis,19 and only one example employing an unnatural AA (Aib).20 As a result, a sample containing 17b, Ac-Ala-OH and EEDQ in THF was irradiated for 30′ at the lowest temperature reachable by a conventional compressed air-cooled MW reactor (80 Watt) (Table 1, entry 6). After 30 minutes, the solution was by cooled and peptide 2b directly precipitated in pure form in 60% yield.
Conformational study
X-ray diffraction
Single crystals of 6a were grown by slow evaporation from a mixture 1
:
1 of ethyl acetoacetate and chloroform, while those of compound 2b by slow evaporation from a solution of acetonitrile (ESI†).
X-ray analysis of 6a. The analysis allowed the determination of the absolute stereochemistry of the five asymmetric carbons (Fig. 2) and evidenced hydrogen bonding interactions (ESI pp. S56†).
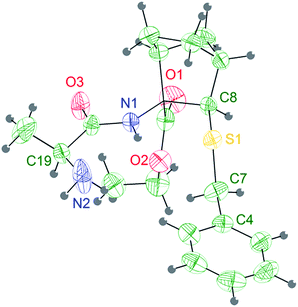 |
| Fig. 2 Stereoview of the molecular structure of compound 6a at 298 K, showing 30% probability displacement ellipsoids. | |
The Ala group undergoes a significant apparent thermal motion, which could imply some kind of disorder. As for the crystal packing (ESI pp. S56†), the system forms zig-zag ribbons of head-to-tail hydrogen bonded (HB) molecules parallel to the a axis. The only relevant HB contact involves one H atom belonging to the terminal amine group and the amidic oxygen of an opposing Ala unit. Another possible HB donor is the amidic N–H group, which is actually involved in an intramolecular short contact with the sulfur atom (Fig. 2 and Table 2).
Table 2 Relevant short H⋯O,S contacts in 6a. Values are given in Å and degrees, with sensible estimated standard deviations reported in parentheses
D–H⋯A |
D–H |
Distance H⋯A |
Distance D⋯A |
Angle D–H⋯A |
Symmetrya |
Symmetry operation generating the acceptor (‘A’) atom. Intramolecular contact. |
N2–H2B⋯O2 |
0.90 |
2.18 |
2.990(4) |
149.3 |
−1/2 + x, 1/2 −y, 1 − z |
N1–HN1⋯S1 |
0.86 |
2.48 |
2.995(2) |
119.0 |
x, y, zb |
X-Ray analyses of 2b. The analysis confirmed the absolute stereochemistry of NRB and that no racemization occurred during the whole synthetic process. Interestingly, the asymmetric unit is composed of two molecules (2bi and 2bii hereinafter) as shown in Fig. 3a and b. The φ and ψ dihedrals of both the conformations present in the crystal cell were close to the ideal values of a 310-helix (φ = −49; ψ = −26) with a right handed screw sense, as shown in Table 3.
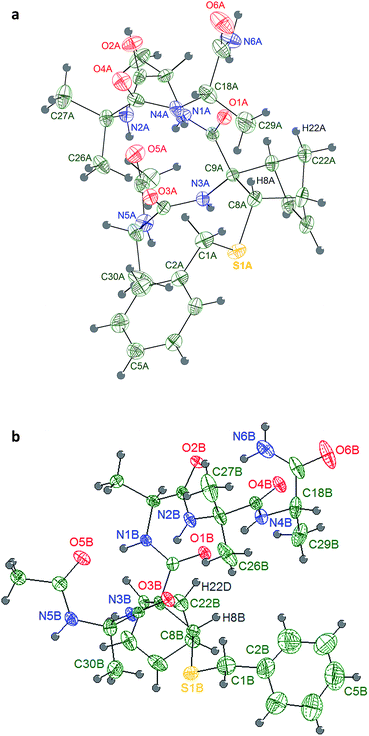 |
| Fig. 3 (a) Stereoview of the molecular structure of compound 2b in the crystal state at 150 K, showing 50% probability displacement ellipsoids. Only the molecule numbered as 2bi in the asymmetric unit is shown. (b) Stereoview of the molecular structure of compound 2b in the crystal state at 150 K, showing 50% probability displacement ellipsoids. Only the molecule numbered as 2bii in the asymmetric unit is shown. | |
Table 3 Dihedral angle simulated and observed in the crystal structurea
|
Simulatedb |
Peptide 2bi |
Peptide 2bii |
2bi and 2bii are the two different conformers of peptide 2b in the crystal cell. Dihedral values obtained by REMD simulation, as described in the computational analysis section. |
ψ0 |
|
−57.4 |
−54.4 |
ψ1 |
−13.61 ± 34.74 |
−32.8 |
−42.0 |
φ1 |
−53.12 ± 16.48 |
−50.0 |
−53.5 |
ψ2 |
−29.37 ± 14.99 |
−32.1 |
−39.9 |
φ2 |
−62.45 ± 18.99 |
−65.3 |
−63.1 |
ψ3 |
−26.30 ± 15.16 |
−17.2 |
−17.9 |
φ3 |
−55.06 ± 9.96 |
−55.5 |
−50.4 |
ψ4 |
−24.25 ± 12.81 |
−42.2 |
−34.8 |
φ4 |
−106.84 ± 35.15 |
−81.6 |
−73.8 |
φ5 |
|
−48.1 |
−20.3 |
Four consecutive intramolecular short contacts occur in both conformers. They involve the NH protons of CONH2, Ala(5)NH, Aib(4)NH and Ala(3)NH, as listed together with their geometrical parameters in Table 4. Three of them are common in the two conformers, i.e. they involve the same NH groups as donors (N1, N2 and N4) and the same O atoms as acceptors (O5, O3 and O1). Again in both the conformers, out of the two NH protons of the CONH2 group, only one is involved in a short intramolecular contact: while in conformer 2bi the interaction arises between the N6A–HN6A2 group and atom O1A, in 2bii it involves the N6B–HN6B2 and atom O2B. The pattern of H bonds underlined by the crystal structure completely reflects the typical distribution i–i + 3 of 310-helix for the conformer 2bii. Regarding 2bi the 310-helix H bond distribution is respected except for the CONH2 proton that present a i–i + 4 hydrogen bond interaction typical of α-helix. Such deviation of 2bi from ideal values for the terminal Ala(5) is also detectable in the angles ψ4 and φ4 that differ much more from ideal 310-helix than in 2bii.
Table 4 Intramolecular H-bond parameters observed for compound 2b in the crystal state. Estimated standard deviations are reported in parentheses. Distances in Å angles in (°)
Conformer |
Group |
Donor D–H |
Acceptor A |
Distance D⋯A |
Distance H⋯A |
Angle D–H⋯A |
2bi |
Ala(3) |
N1A–HN1A |
O5A |
2.939(4) |
2.116(3) |
159.8(2) |
2bii |
N1B–HN1B |
O5B |
3.003(4) |
2.277(3) |
142.2(2) |
2bi |
Aib(4) |
N2A–HN2A |
O3A |
2.960(3) |
2.134(2) |
160.9(2) |
2bii |
N2B–HN2B |
O3B |
2.983(4) |
2.176(2) |
156.0(2) |
2bi |
Ala(5) |
N4A–HN4A |
O1A |
2.984(4) |
2.284(3) |
138.6(2) |
2bii |
N4B–HN4B |
O1B |
3.032(4) |
2.206(2) |
160.9(2) |
2bi |
CONH2 |
N6A–HN6A2 |
O1A |
3.141(4) |
2.288(2) |
171.8(2) |
2bii |
N6B–HN6B2 |
O2B |
2.910(4) |
2.122(3) |
151.9(2) |
2bi |
NRB(2) |
C8A–H8A |
O1A |
2.796(4) |
2.274(3) |
112.3(2) |
2bii |
C8B–H8B |
O1B |
2.851(4) |
2.365(3) |
109.9(2) |
2bi |
NRB(2) |
C22A–H22A |
O1A |
3.131(4) |
2.453(4) |
126.6(2) |
2bii |
C22B–H22D |
O1B |
3.154(4) |
2.480(3) |
126.4(2) |
Weak hydrogen interactions (i.e. C–H⋯O, C–H⋯N and C–H⋯π)22 have been found to be critical in stabilizing different molecular geometries.23–28 In a previous work, our computational model underlined that the formation of an intra-residue C–H⋯O hydrogen bond was responsible of the helix stabilization exerted by an unsubstituted norbornene AA included in the same peptide model herein described.16 In both 2bi and 2bii, it can be noticed the presence of a C–H⋯O interaction between H22A and O1A, corresponding to that observed in the computational analysis of the unsubstituted norbornene, but also an additional interaction between H8A and O1A (Table 4), which might further enhance the NRB helix stabilizing power thanks to the correct orientation of ψ2.
Moreover, to our knowledge, only few example of crystal structures of pentapeptides containing both proteogenic and non proteogenic AAs have been reported so far containing at least two non proteogenic AAs.21 The obtainment of a well-defined crystal structure for a pentapeptide containing only two non-proteogenic AAs, Aib and NRB, suggests that the pentapeptide is strongly structured.
NMR spectroscopy
Both peptides 2a and 2b were subjected to NMR conformational analysis. Experiments were performed in both cases in CD3CN (20 mM) in order to avoid the hydrophobic collapse typical of chlorinated solvent and simulate as much as possible a polar medium. Homonuclear and heteronuclear 2D NMR experiments permitted an overall assignment of 2a and 2b proton and carbon resonances (see ESI, pp. S4–S12,† COSY, HSQC, HMBC spectra).
NOESY experiments on compound 2b. NOESY spectra allowed the identification of all sequential short range NHi–NHi+1 cross peaks in the amide region (ESI, pg. S8†), that are diagnostic of helical conformation.29The analysis of Cα(β)H–NH enabled the discrimination between the 310-helix and the α-helix structures. No CαHi–NHi+4 cross-peaks inherent of α-helix were detectable in the NOESY spectra, but many diagnostic CαHi–NHi+3 and CαHi–NHi+2 medium range cross-peak signals typical of 310-helix were present. An inspection of the fingerprint area of NOESY spectrum revealed three CαHi–NHi+3 [Ala(1)Hα–Aib(4)NH, Ala(3)Hα–CONH2 (Fig. 4) and AcMe–Ala(3)NH, (Fig. 5)], and two CαHi–NHi+2 [Ala(1)Hα–Ala(3)NH (Fig. 4), AcMe–NRB(2)NH (Fig. 5)] supporting the hypothesis of a 310-helix structure.
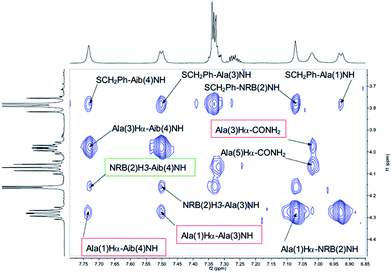 |
| Fig. 4 CαH–NH cross peaks in the NOESY spectrum (τm 500 ms, BBI probe, 300 K, 500 MHz) of peptide 2b in CD3CN (20 mM). Sequential short range CαHi–NHi+1 signals diagnostic for structured peptide. Medium range CαHi–NHi+3 and CαHi–NHi+2 signals typical of 310-helix (α red box, β green box). | |
 |
| Fig. 5 CβH–NH cross peaks in the NOESY spectrum (τm 500 ms, BBI probe, 300 K, 500 MHz) of peptide 2b in CD3CN (20 mM). Medium range CβHi–NHi+3 and CβHi–NHi+2 diagnostic for helical structure (α red box, β green box). | |
Also medium and short range signals CβHi–NHi+3 (Ala(1)Me–Aib(4)NH, Ala(3)Me–CONH2 and Aib(4)Me–Ala(1)NH, Fig. 6) and CβHi–NHi+2 [Ala(5)Me–Ala(3)NH, Aib(4)Me–CONH2 (Fig. 5) and NRB(2)H3–Aib(4)NH (Fig. 4)] confirmed the initial hypothesis.
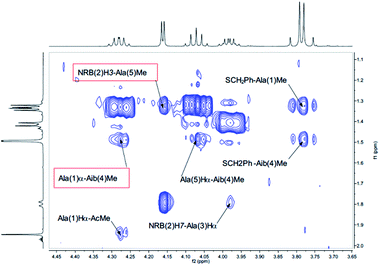 |
| Fig. 6 αH–Me of the NOESY spectrum (τm 5000 ms, BBI probe, 300 K, 500 MHz) of peptide 2b in CD3CN (20 mM). | |
Finally, a high degree of structuration of peptide 2b could be inferred by the presence of an unusual and intense long range cross-peak between the H3 proton of the norbornene scaffold and the Me groups of Ala(5) and Ala(1)α–Aib(4)Me as shown in Fig. 6.
NOESY experiments on compound 2a. Despite the overlap of two Hα and two NH amide protons which complicated the interpretation of NMR data, valuable structural information were obtained. Using standard sample conditions in CD3CN (20 mM) all sequential short range NHi–NHi+1 interactions were visible (ESI, pg. S13†) except the interaction between Ala(5)NH–CONH2, obscured by the phase of diagonal peaks. By acquiring the NOESY spectrum at lower concentration (8 mM), we identified the entire sequence confirming an helical construct for 2a (ESI, pg. S13†).23Moreover, 3D structural information present in the Cα(β)H–NH region of the NOESY spectrum of 2a were enough to confirm the preference of a 310-helix over a α-helix. Detectable interactions Cα(β)Hi–NHi+2 (Ala(1)Hα–Ala(3)NH, NRB(2)Hβ–Aib(4)NH, AibMe–CONH2 and AcMe–NRB(2)NH) and CαHi–NHi+3 (Ala(1)Hα–Aib(4)NH, NRB(2)Hβ–Ala(5)NH and AcMe–Ala(3)NH) are shown in Fig. 7 and 8. In conclusion, the absence of C α(β)Hi–NHi+4 cross peaks in the NOE spectrum suggests that both enantiomers of 1, once inserted in the model peptides 2a and 2b, stabilize a 310-helical structure.
 |
| Fig. 7 CαH–NH cross peaks in the NOESY spectrum (τm 500 ms, BBI probe, 300 K, 500 MHz) of peptide 2a in CD3CN (20 mM). Sequential short range CαHi–NHi+1 signals diagnostic for structured peptide. Medium range Cα(β)Hi–NHi+3 and Cα(β)Hi–NHi+2 signals typical of 310-helix (α red box, β green box). | |
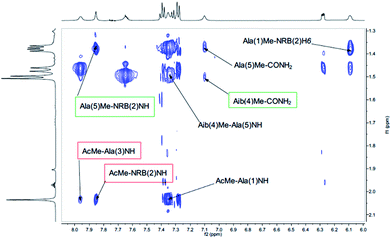 |
| Fig. 8 CβH–NH of the NOESY spectrum (τm 500 ms, BBI probe, 300 K, 500 MHz) of peptide 2a in CD3CN (20 mM). Sequential short range CαHi–NHi+1 signals diagnostic for structured peptide. Medium range Cα(β)Hi–NHi+3 and Cα(β)Hi–NHi+2 signals typical of 310-helix (α red box, β green box). | |
VT-NMR and DMSOd6 titration. To confirm the presence of a 310-helical structure, the H-bond network within the peptide backbone was analyzed by measuring the temperature coefficient of the chemical shifts (Δδ(NH)/ΔT) for each NH of 2a and 2b, as well as the variation of chemical shift by DMSO-d6 titration (Δδ/ΔDMSO-d6 v/v).Three stable consecutive H-bonds, involving NH protons of CONH2, Ala(5)NH and Aib(4)NH, are detected (Fig. 9 and 10) demonstrating, according to our proposal, a full helical construct for both peptides 2a and 2b. In peptide 2b, Ala(3)NH also presents very low Δδ values, supporting the presence of a further intramolecular H-bond which is only compatible with 310-helical structure. Indeed, the N terminal Ala(1)NH coefficient and, as might be expected, one NH proton of CONH2 group are near to the limit of −4.5 ppb K−1 that points out the not H-bonded NH protons. The DMSO-d6 titration reflects the result of VT-NMR, since no variation of chemical shift are detectable for one NH proton of CONH2 group, Ala(5)NH, Aib(4)NH and Ala(3)NH endorsing the presence of four H-bonds typical of 310-helix (Fig. 10). In both experiments related to 2a and 2b, the NRB(2)NH amide proton showed an unexpected stability. Considering that NH solvent exposition influences, to varying degrees, both the variable-temperature analysis and DMSO-d6 titration, such result could be a false positive ascribed to the solvent exclusion sphere generated by the norbornene scaffold and the benzylsulfanyl group, as supported by NOESY spectra. The same hypothesis could also explain the high Δδ observed for Ala(3)NH in 2a, which disagrees with the extremely stable trend found in DMSO-d6 titration.
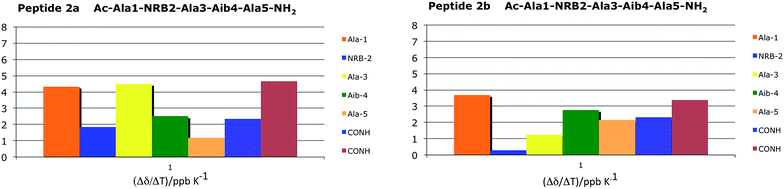 |
| Fig. 9 Temperature coefficients for amide protons chemical shifts of 2a and 2b (CD3CN 10 mM) in a range temperature of 273–335 K. | |
 |
| Fig. 10 Chemical shift variation for amide proton in function of DMSO-d6 v/v% of 2a and 2b (CD3CN 10 mM) in a range of 0–22 v/v%. | |
Non-magnetic equivalence. Lastly, a proof of an high content of a preferred helical conformation could be deduced from the anisochronicity of the 13C NMR signals of the diastereotopic methyl groups in Aib(4). Considering that neighboring chiral residues could not induce a non-magnetic equivalence (NME) higher than 0.5 ppm, the large NME with value of 3.07 ppm (26.26–23.19 ppm, ESI, pg. S14†) for peptide 2b and 3.88 ppm (26.60–22.72 ppm, ESI, pg. S15†) for peptide 2a could only derive from the presence of a stable helical secondary structure.30
Computational analysis
In order to gain a deeper knowledge about the folding preferences of peptides 2a and 2b, based on the two enantiomers (R,R,R,S) and (S,S,S,R)-NRB, we planned a molecular dynamics (MD) study of the two pentapeptides models using the Amber12 package.31 The replica exchange molecular dynamics (REMD) method,32 a generalized-ensemble algorithm performing random walks in energy space, thus helping a system to escape from local energy traps, has been successfully adopted in secondary structure predictions due to its improved sampling capabilities over standard MD runs. REMD simulations (12 replicas with temperatures exponentially spaced between 260 and 660 K) were then performed by starting from extended conformations and by using a protocol previously optimized for similar questions.6b The peptide was simulated for 50 ns, and analyses were performed on the final 25 ns of the 308.5 K trajectory, being this temperature the closest one to experimental conditions. Trajectories were analyzed in terms of H-bonds, secondary structure and cluster analyses (Tables 5–7). Although the adopted simulation protocol already proved to be successful in predicting the structure of similar peptide models containing CTAAs,6b the comparison of the representative structure obtained from a cluster analysis of the REMD trajectory of peptide 2b with the corresponding crystal structure further supported the reliability of the method adopted here (Fig. 11 and Table 3).
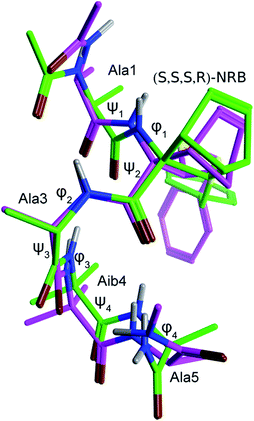 |
| Fig. 11 Comparison between the X-ray structure of 2b (carbon colored in green) and the representative geometry of the most populated cluster coming from the cluster analysis of the 308.5 K REMD trajectory of 2b (RMSD (Cα) = 1.1 Å). | |
As we can see from H-bond analysis (Table 5), both peptides 2a and 2b are characterized by two i + 3 → i H-bonds, which are typical of 310-helices, between residues 1–4 (Ala(1) and Aib(4), respectively) and 2–5 (NRB(2) and Ala(5), respectively). Although the overall H-bond occupancy in 2a and 2b is comparable, it can be observed that the most stable H-bond is found between Ala(1) and Aib(4) (occupancy = 68.4%) in 2a, while in 2b it is between NRB(2) and Ala(5). In both structures, the third observed H-bond is of the i + 4 → i type, indicative of an α-helix, and has an occupancy of 2.5 and 1.6%, respectively, suggesting that this secondary structure is only occasionally sampled.
Table 5 H-bonda analysis of the last 25 ns of the 308.5 K ff99SB REMD trajectory for peptides 2a and 2b
H-bonds |
2a (R,R,R,S)-NRB |
2b (S,S,S,R)-NRB |
Occupancy |
Only H-bonds with an occupancy >1% are reported. H-bonds involving the capping Ac and NHMe groups are not reported. |
Ala(1) C O⋯HN Aib(4) |
68.4% |
46.4% |
Ala(1) C O⋯HN Ala(5) |
2.5% |
1.6% |
NRB(2) C O⋯HN Ala(5) |
54.2% |
73.4% |
The secondary structure analysis (Table 6), based on the DSSP method,33 while confirming the results of H-bond analysis, provided per-residue information on the secondary structure. Interestingly, as also observed from the H-bond analysis, (R,R,R,S)-NRB seems to propagate the 310-helix stabilization toward the N-terminus, while the (S,S,S,R)-NRB enantiomer seems to exert its stabilizing effect mostly toward the C-terminus of the model peptide.
Table 6 Secondary structure analysisa of the last 25 ns of the 308.5 K ff99SB REMD trajectory for peptides 2a and 2b
|
2a (R,R,R,S)-NRB |
2b (S,S,S,R)-NRB |
310-helix |
α-helix |
Turn |
310-helix |
α-helix |
Turn |
Values are reported as a percentage of the total. The difference to 100% is the percentage of unordered secondary structure. |
Ala(1) |
67.2 |
5.8 |
12.3 |
32.3 |
1.8 |
9.6 |
NRB(2) |
79.7 |
6.1 |
13.2 |
47.3 |
1.9 |
10.8 |
Ala(3) |
82.5 |
6.1 |
10.7 |
71.1 |
1.9 |
18.6 |
Aib(4) |
66.2 |
6.4 |
24.6 |
67.8 |
2.0 |
25.4 |
Ala(5) |
44.1 |
1.3 |
31.4 |
52.6 |
0.4 |
31.4 |
Further confirmation came from the cluster analysis of the REMD trajectories. As reported in Table 7, the first three most populated cluster represent over the 95% of the total population for both 2a and 2b peptides. Concerning 2a, φ and ψ values for the representative structure of cluster #1 (pop. = 79.1%) fall within the helix region, with a quite significant deviation only for the φ4 dihedral angle of the C-terminal Ala(5). A quite wide ψ2 dihedral angle is also observed (−57.7 deg.), possibly due to the steric hindrance between the –SBn group of NRB(2) and the –CH3 of Ala(3) (C3′HH–CH2– distance = 2.5 Å); (see figure reported in the ESI, pg S52†) cluster #2, which is significantly populated (pop. = 20.3%), corresponds to a partially folded structure where φ and ψ values for residues 1–3 fit with a right-handed helix, while Aib(4) φ and ψ dihedrals angles fall in the left-handed helix region, analogously to what observed for other CTAAs.6b,16 Clusters #3–5 are barely populated (pop. = 0.3, 0.3 and 0.0%, respectively) and their representative structures correspond to unordered conformations. Concerning peptide 2b, cluster #1 (pop. = 82.6%) also corresponds to a well ordered 310-helix and, differently from what observed for 2a, the ψ2 dihedral angles also fits with such a secondary structure. Indeed, due to the opposite stereochemical configuration, the –SBn group of NRB(2) points away from the helix core (Fig. S8 and pg S53, ESI†). Cluster #2, 8.7% of the total population, represents a folding intermediate with most of the φ and ψ dihedral angles falling in the left-handed helix region, while, interestingly, the representative structure of cluster #3 (pop. = 6.0%), corresponds to a completely folded left-handed helix (Fig. S6, page S55 ESI†), a secondary structure not observed for 2a (figure reported in the ESI, pg S52†). Clusters #4 and #5, overall representing 2.7% of the total population, correspond to an unfolded conformation and a left-handed folding intermediate, respectively. Taken together, in agreement with the experimental result, the above data confirm that both (R,R,R,S)- and (S,S,S,R)-NRB enantiomers possess a strong helicogenic effect when inserted in short Ala-Aib sequences. In our previous work on the Azn CTAA6b only the (R)-enantiomer was shown to be helicogenic within Ala-Aib pentapeptides, suggesting that the rigid norbornane core plays a positive effect on the ability to induce helical secondary structures. However, considering the results from REMD simulations and cluster analyses, it cannot be excluded that the (S,S,S,R)-NRB enantiomer could also behave as a powerful inducer of left-handed helices if coupled with D-AAs and/or achiral CTAAs such as Aib.
Table 7 Geometrical parameters for the most representative structures deriving from the cluster analysisa of the final 25 ns of the 308.5 K ff99SB REMD trajectory for peptides 2a and 2b. Cluster populations (%) are reported in parenthesis
|
2a (R,R,R,S)-NRB |
2b (S,S,S,R)-NRB |
#1 (79.1) |
#2 (20.3) |
#1 (82.6) |
#2 (8.7) |
Results for the two most populated clusters, covering above 90% of the total population, are reported. Populations (%) of the remaining 3 clusters are: 0.3, 0.3, and 0.0 for 2a, 6.0, 1.9, 0.8 for 2b. Geometries of the most representative structures of 2a and 2b are depicted in figures reported in the ESI, pp. S52 and S53, respectively. |
ψ1 |
−14.2 |
−46.5 |
−17.6 |
25.4 |
φ1 |
−43.1 |
−56.6 |
−48.0 |
66.6 |
ψ2 |
−57.7 |
−27.1 |
−14.2 |
31.2 |
φ2 |
−43.3 |
−126.0 |
−35.9 |
−154.1 |
ψ3 |
−20.2 |
18.4 |
−43.1 |
8.2 |
φ3 |
−48.3 |
66.3 |
−47.3 |
58.8 |
ψ4 |
−46.4 |
1.8 |
−26.5 |
14.3 |
φ4 |
−121.1 |
−77.2 |
−144.5 |
−115.7 |
Conclusions
We have prepared two model pentapeptides 2a and 2b containing the unnatural cysteine mimic, 3-benzylsulfanylnorbornene amino acid (NRB) in a short chain sequence of Ala/Aib. All the synthetic steps were optimized allowing the preparation of a satisfactory amount of the pentapeptides containing steric congested NRB in good yields with a new MW assisted protocol. The presence of the C5–C6 double bond in the norbornene scaffold opens the way to further functionalization and/or reduction directly on the model peptides. The conformation of the model compounds 2a and 2b was studied by both theoretical calculation and spectroscopic analyses and confirmed by X-ray analysis. Interestingly, all the collected data confirm that both NRB enantiomers possess a strong right-handed helicogenic effect in the presence of L-alanine, as previously observed for the unsubstituted norbornene AA, where this peculiar effect was explained by the network of weak hydrogen interactions stabilized by the rigid norbornane core.16 Moreover crystal structure gave a proof of the stabilization mechanism of such core. These results open the way for the design and the synthesis of stable peptides able to modulate pharmacologically relevant PPIs.
Experimental
Synthetic procedures
Compounds 1 and 5 have been previously described.10
(1S,2R,3R,4R)-Ethyl 2-[(S)-2-aminopropanamido-3-(benzylthio)bicyclo[2.2.1]heptane-2-carboxylate (6a) and (1R,2S,3S,4S)-ethyl 2-[(S)-2-aminopropanamido-3-(benzylthio)bicyclo[2.2.1] heptane-2-carboxylate (6b). To a solution of 5 (518 mg, 1.7 mmol) in dry DCM (10 mL), BSA (138 mg, 6.8 mmol) was added and let to react over-night at room temperature under stirring. FmocAlaF (638.5 mg, 2.04 mmol) was added and then the reaction mixture was made to react at room temperature for 20 minutes. The reaction was monitored by Tlc (AcOEt
:
n-hexane, 1
:
2; detected by ninhydrin). At the end of the reaction, the solvent was evaporated at reduced pressure and the crude purified by flash chromatography (eluent AcOEt
:
n-hexane, 1
:
6 to 1
:
2) affording the Fmoc derivatives in mixture (white solid, 85% yield) which were directly subjected to deprotection. The mixture was dissolved in the minimum amount of DMF (3 mL) and piperidine (150 μL) was added. The mixture was stirred at room temperature until disappearance of the reactants (1 h, Tlc AcOEt
:
n-hexane, 1
:
2; detected by ninhydrin). The solvent was evaporated at reduced pressure and the crude purified by flash chromatography (eluent DCM
:
MeOH, 100
:
0 to 50
:
1) affording small amounts of compound 6a and 6b (yellow solid, 95% overall yield) in pure form together with a major amount of their mixture.
6a. Mp 351–352 °C, αMeOHD = −85.95. 1H-NMR δ (200 MHz, CDCl3): 8.62 (1H, s), 7.35–7.21 (5H, m), 4.26–4.10 (2H, m), 3.91–3.74 (2H, br s), 3.66–3.56 (1H, m), 3.21–3.16 (2H, m), 2.30–2.15 (2H, s), 1.94–1.88 (1H, d, J = 11 Hz), 1.48–1.21 (11H, m); 13C-NMR δ (50 MHz, CDCl3): 175.21, 173.59, 137.84, 129.13, 128.81, 127.51, 62.29, 61.40, 56.35, 51.18, 44.25, 42.41, 37.98, 36.55, 23.59, 22.75, 21.69, 14.37. (+)ESI-MS (m/z): 377.1 [M + H]+. Anal. calcd for C20H28N2O3S (376.1): C, 63.80; H, 7.50; N, 7.44; found C, 63.30; H, 7.38; N, 7.20; IR (KBr): ν = 3296, 2964, 1737, 1669 cm−1.
6b. Mp 273–276 °C, αMeOHD = +117.75 1H-NMR δ (200 MHz, CDCl3): 8.60 (1H, s), 7.32–7.23 (5H, m), 4.24–4.16 (2H, m), 3.90–3.76 (2H, br s), 3.60–3.54 (1H, m), 3.21–3.17 (2H, m), 2.32 (1H, s), 1.92–1.87 (1H, d, J = 11 Hz), 1.65 (2H, s), 1.48–1.22 (11H, m); 13C-NMR δ (50 MHz, CDCl3): 175.61, 173.76, 137.93, 129.12, 128.76, 127.46, 62.32, 61.40, 56.45, 51.31, 44.21, 42.46, 38.03, 36.50, 23.47, 22.72, 22.09, 14.36. (+)ESI-MS (m/z): 377.1 [M + H]+. Anal. calcd for C20H28N2O3S (376.1): C, 63.80; H, 7.50; N, 7.44; found C, 64.15; H, 7.55; N, 7.18; IR (KBr): ν = 3298, 2963, 1733, 1668 cm−1.
(1R,2R,3R,4S)-Ethyl 2-((S)-2-acetamidopropanamido)-3-(benzylthio)bicyclo[2.2.1]heptane-2-carboxylate (7a). Compound 6a (102 mg, 0.270 mmol) was dissolved in dry DCM (5 mL) under nitrogen and TEA (56.48 μL, 0.406 mmol) and acetic anhydride (38.34 μL, 0.406 mmol) were added under stirring at room temperature. The mixture was made to react until disappearance of the reactants (2 h, Tlc DCM
:
MeOH, 10
:
1; detected by ninhydrin). The organic phase was washed with HCl (0.1 N), saturated NaHCO3 and then was dried over Na2SO4 and the solvent evaporated at reduced pressure to afford the acetylated ester (yellow oil, 75% yield) which was directly submitted to ester hydrolysis. The crude (83 mg) was dissolved in DCM
:
MeOH (9
:
1, 2 mL) and a methanolic solution of NaOH (201 μL, 0.64 mmol) was added. The mixture was heated (50 °C) until disappearance of the reactants (12 h, Tlc DCM
:
MeOH, 10
:
1). The solvent was evaporated at reduced pressure and the crude extracted with diethyl ether. The aqueous phase was taken up and cooled to 0 °C before adding HCl 1 N to pH = 2. The acidic aqueous phase was extracted with cold AcOEt, the organic phase was dried over Na2SO4 and the solvent evaporated at reduced pressure to afford a mixture containing 6a as the major compound together with the diastereisomeric compound containing the R-alanine.
7a. 1H-NMR δ (200 MHz, CD3OD): 8.14 (1H, s), 7.34–7.19 (5H, m), 4.53–4.43 (1H, m), 3.87–3.73 (2H, m), 3.16 (1H, s), 3.06 (1H, s), 2.21 (1H, s), 1.99 (3H, s), 1.92–1.87 (1H, m), 1.46–1.18 (8H, m). 13C-NMR δ (50 MHz, CD3OD): 176.88, 174.20, 173.79, 137.93, 129.17, 129.11, 127.86, 64.91, 51.35, 49.15, 46.09, 42.65, 36.95, 35.82, 22.93, 21.07, 16.23, 15.46. (+)ESI-MS (m/z): 377.3 [M − COMe]+. Anal. calcd for C20H30N2O4S (418.55): C, 63.13; H, 7.22; N, 6.69; found C, 63.40; H, 7.27; N, 6.30; IR (KBr): ν = 3435, 2850, 1721, 1638 cm−1.
(S)-tert-Butyl(1-((1-amino-1-oxopropan-2-yl)amino)-2-methyl-1-oxopropan-2-yl)carbamate (10). To a solution of BocAibOH 8 in dry DCM (0.1 M), HOAt (1.1 eq.) and EDC (1.1 eq.) were added under nitrogen at 0 °C and let to react under stirring at 0 °C for 1 h. After that, l-AlaCONH2 9 (1.1 eq.) and DIPEA (2.2 eq.) were added, followed by DIPEA to reach pH = 8, and then the reaction mixture was made to react at room temperature for 12 h. The reaction was monitored by Tlc (MeOH
:
DCM, 1
:
10; detected by ninhydrin). Upon the consumption of the starting material the reaction mixture was washed with saturated NH4Cl, saturated NaHCO3, and brine. The organic phase was dried over Na2SO4 and the solvent evaporated at reduced pressure to afford compound 10 as white wax (75% yield). The reaction was performed with comparable yield from 0.1 g to 2 g. αMeOHD = −7.6. 1H-NMR δ (200 MHz, CDCl3): 7.08 (1H, s), 6.53 (1H, d, J = 7.2 Hz), 5.34 (1H, s), 5.01 (1H, s), 4.54–4.39 (1H, m), 1.79–1.40 (18H, m); 13C-NMR δ (50 MHz, CDCl3) 175.04, 174.25, 155.47, 81.14, 57.02, 49.09, 28.46, 26.63, 24.75, 17.85. (+)ESI-MS (m/z): [M + Na]+ 296.1. Anal. calcd for C12H23N3O4 (273.17): C, 52.73; H, 8.48; N, 15.37; found C, 52.78; H, 8.53; N, 15.40. IR (KBr): ν = 3400, 3370, 2984, 1693, 1673, 1515 cm−1.
(S)-2-Amino-N-(1-amino-1-oxopropan-2-yl)-2-methylpropanamide (11). Trifluoroacetic acid 99% (TFA, 30 eq.) was added dropwise under stirring to a solution of compound 10 (1 eq.) in dry DCM (0.1 M) at 0 °C. The solution was warmed up at room temperature and let to react for 4 hours. The reaction was monitored by Tlc (MeOH
:
DCM, 1
:
10; detected by ninhydrin). Upon consumption of the starting material the solvent was evaporated at reduced pressure to afford the desired compound 11 as light yellow oil (92% yield). The reaction was performed with comparable yield from 0.1 g to 2 g. Mp 254 °C, αMeOHD = +4.28. 1H-NMR δ (200 MHz, CD3OD): 4.43–4.32 (1H, m), 1.59 (6H, d, J = 5.19 Hz), 1.41 (3H, d, J = 11.54 Hz); 13C-NMR δ (50 MHz, CD3OD): 175.98, 171.65, 57.01, 49.35, 22.94, 22.81, 16.85. (+)ESI-MS (m/z): [M + Na]+ 174.1. Anal. calcd for C9H16F3N3O4 (173.12 + 114): C, 37.63; H, 5.61; N, 14.63; found C, 37.68; H, 5.58; N, 14.67; IR (NaCl): ν = 3418, 2095, 1673, 1545, 1204 cm−1.
tert-Butyl((S)-1-((1-(((S)-1-amino-1-oxopropan-2-yl)amino)-2-methyl-1-oxopropan-2-yl)amino)-1-oxopropan-2-yl)carbamate (13). To a solution of Boc-(l)AlaOH 12 in dry DCM (0.1 M), HOAt (1.1 eq.) and EDC (1.1 eq.) were under nitrogen at 0 °C and let to react under stirring at 0 °C for 1 h. After that, 11 (1.1 eq.) and DIPEA (2.2 eq.) were added, followed by additional DIPEA to reach pH = 8, and then the reaction mixture was made to react at room temperature for 12 h. The reaction was monitored by Tlc (MeOH
:
DCM, 1
:
10; Rf 0.19, detected by ninhydrin). Upon the consumption of the starting material the reaction mixture was washed with saturated NH4Cl, saturated NaHCO3, and brine. The saturated NH4Cl solution of the first washing was re-extracted 3 times with EtOAc and the combined organic phases dried over Na2SO4. The solvent was evaporated at reduced pressure and the crude purified by flash chromatography (eluent MeOH
:
DCM, 1
:
10 to 1
:
5) to afford compound 13 as white wax (69% yield). The reaction was performed with comparable yield from 0.1 g to 2 g. αMeOHD = −20. 1H-NMR δ (200 MHz, CD3OD): 4.21 (1H, q, J = 7.3 Hz), 3.88 (1H, q, J = 7.3 Hz), 1.60–1.39 (18H, m), 1.30 (3H, d, J = 7.3 Hz). 13C-NMR δ (50 MHz, CD3OD): 177.00, 175.50, 175.31, 157.18, 79.88, 56.54, 51.96, 49.76, 27.53, 25.34, 23.32, 16.53, 16.02. (+)ESI-MS (m/z): [M + Na]+ 367.2. Anal. calcd for C15H28N4O5 (344.4): C, 52.31; H, 8.19; N, 16.27; found C, 52.36; H, 8.24; N, 16.31. IR (KBr): ν = 3410, 3310, 2981, 2938, 1679, 1665, 1536 cm−1.
N-((S)-1-Amino-1-oxopropan-2-yl)-2-((S)-2-aminopropanamido)-2-methylpropanamide (4) (HAla-Aib-AlaNH2). Compound 13 was dissolved in water (0.1 M) in a sealed tube for microwaves reactor. The solution was irradiated by microwaves under magnetic stirring for 20 min at 150 °C. The reaction was monitored by Tlc (MeOH
:
DCM, 1
:
10; detected by ninhydrin). If not finished, another round of 20 minutes was performed. Upon consumption of the starting material, water was evaporated at reduced pressure to afford the desired compound 4 as light yellow solid (94% yield). The reaction was performed with comparable yield from 0.1 g to 2 g. αMeOHD = −1.55. 1H-NMR δ (200 MHz, CD3OD): 4.30 (1H, q, J = 7.2 Hz), 3.44 (1H, q, J = 6.9 Hz), 1.46–1.26 (12H, m); 13C-NMR δ (50 MHz, CD3OD): 176.70, 176.67, 175.32, 56.32, 50.38, 49.28, 24.48, 23.86, 19.65, 16.54. (+)ESI-MS (m/z): [M + Na]+ 244.15. Anal. calcd for C10H20N4O3 (267.3): C, 49.17; H, 8.25; N, 22.93; found C, 49.22; H, 8.29; N, 22.98. IR (KBr): ν = 3399, 3064, 2985, 1660, 1530 cm−1.
(1R*,2R*,3R*,4S*)-Ethyl-3-(benzylthio)-2-((tert-butoxycarbonyl)amino bicyclo[2.2.1]hept-5-ene-2-carboxylate (14). To solution of compound 1 in dry DCM (0.1 M), Boc-anhydride (2 eq.) followed by dry TEA (2 eq.) were added at 0 °C and let to react at rt for 4 h. The reaction is monitored bt Tlc (EtOAc
:
hexane, 1
:
2; detected by ninhydrin). Upon the consumption of the starting material the crude mixture was diluted with DCM and washed with HCl 1 M, saturated NaHCO3, and brine. The organic phase was dried over Na2SO4 and the solvent was evaporated at reduced pressure. The crude product was purified by flash chromatography (eluent EtOAc
:
hexane, from 1
:
6 to 1
:
2) affording 14 in pure form as colorless oil (90% yield). The reaction was performed with comparable yield from 0.1 g to 2 g.1H-NMR δ (200 MHz, CDCl3): 7.30 (5H, bs), 6.19–6.10 (2H, m), 5.84 (1H, s), 4.26–4.19 (2H, m), 3.79 (2H, s), 3.65 (1H, s), 3.49 (1H, s), 2.89 (1H, s), 1.82 (1H, d, J = 9.3 Hz), 1.58–1.46 (10H, m), 1.29 (3H, t, J = 7.1 Hz). 13C-NMR δ (50 MHz, CDCl3): 174.06, 154.98, 138.01, 137.27, 136.69, 129.10, 128.77, 127.40, 79.69, 64.09, 61.56, 56.51, 50.41, 48.55, 47.39, 37.15, 28.55, 14.39. (+)ESI-MS (m/z): [M + Na]+ 426.2. Anal. calcd for C22H29NO4S (403.3): C, 65.48; H, 7.24; N, 3.47; found C, 65.53; H, 7.20; N, 3.42; IR (KBr): ν = 3358, 2979, 1738, 1714, 1530 cm−1.
(1R*,2R*,3R*,4S*)-3-(Benzylthio)-2-((tert-butoxycarbonyl)amino)bicyclo[2.2.1]hept-5-ene-2-carboxylic acid (15). To solution of compound 14 in dry DCM
:
MeOH (9
:
1, 0.1 M) in a sealed reactor, a solution of KOH in MeOH 6 M (20 eq.) was added and let to react at 70 °C for 8 h. The reaction is monitored bt Tlc (EtOAc
:
hexane, 1
:
2; detected by ninhydrin and UV light). Upon the consumption of starting material the reaction mixture was filtered, the organic solvent was evaporated and the crude was dissolved in HCl 1 M at 0 °C until pH = 2. Then it was extracted 3 times in EtOAc. The combined organic phases was dried over Na2SO4 and the solvent was evaporated at reduced pressure affording 15 in pure form as colorless wax (88% yield). The reaction was performed with comparable yield from 0.1 g to 1.0 g. 1H-NMR δ (200 MHz, CDCl3): 7.37–7.23 (5H, m), 6.25–6.20 (1H, m), 6.14 (1H, s), 6.02–6.00 (1H, m), 4.16 (1H, d, J = 3.1 Hz), 3.74 (1H, s), 3.67 (2H, s), 3.02 (1H, s), 1.69 (1H, d, J = 9.6 Hz), 1.54 (1H, d, J = 9.8 Hz), 1.47 (9H, s). 13C-NMR δ (50 MHz, CDCl3): 174.61, 158.59, 138.01, 137.26, 135.74, 129.12, 128.89, 127.51, 82.04, 65.05, 55.68, 50.25, 48.82, 46.05, 37.75, 28.46. (+)ESI-MS (m/z): [M + Na]+ 398.2. Anal. calcd for C20H25NO4S (375.15): C, 63.97; H, 6.71; N, 3.73; found C, 63.92; H, 6.65; N, 3.77; IR (KBr): ν = 3339, 2965, 1715, 1485, 1394 cm−1.
tert-Butyl((1R,2R,3R,4S)-2-(((S)-1-((1-(((S)-1-amino-1-oxopropan-2-yl)amino)-2-methyl-1-oxopropan-2-yl)amino)-1-oxopropan-2-yl)carbamoyl)-3-(benzylthio)bicyclo[2.2.1]hept-5-en-2-yl)carbamate (16a).
tert-Butyl((1S,2S,3S,4R)-2-(((S)-1-((1-(((S)-1-amino-1-oxopropan-2-yl)amino)-2-methyl-1-oxopropan-2-yl)amino)-1-oxopropan-2-yl)carbamoyl)-3-(benzylthio)bicyclo[2.2.1]hept-5-en-2-yl)carbamate (16b). To a solution of 15 in dry DCM (0.1 M), HOAt (1.1 eq.) and EDC (1.1 eq.) were added under nitrogen at 0 °C and let to react under stirring at 0 °C for 1 h. After that, 4 (HAla-Aib-AlaNH2) (1.1 eq.) and DIPEA (2.2 eq.) were added, followed by additional DIPEA to reach pH = 8 and the reaction mixture was made to react at room temperature for 8 h. The reaction was monitored by Tlc (MeOH
:
DCM, 1
:
10; detected by ninhydrin or UV light). Upon the consumption of the starting material the reaction mixture was washed with saturated NH4Cl, saturated NaHCO3, and brine. The NH4Cl saturated solution was extracted 3 times with EtOAc. The combined organic phases were dried over Na2SO4 and the solvent evaporated at reduced pressure. The crude was purified by flash chromatography (GraceResolv silica cartridges, eluent DCM
:
MeOH, 120
:
1 to 10
:
1) affording pure compounds 16a and 16b. The reaction was performed with comparable yield from 0.1 g to 1 g.16a (white solid, Mp = 101 °C); yield: 37%. αMeOHD = +50. 1H-NMR δ (200 MHz, CDCl3): 7.57–7.55 (2H, m), 7.39–7.23 (6H, m), 6.72 (1H, d, J = 2.5 Hz), 6.32 (1H, dd, J = 5.5, 2.9 Hz), 6.07 (1H, dd, J = 5.5, 3.1 Hz), 5.94 (1H, s), 5.86 (1H, s), 4.43 (1H, dq, J = 7.7, 7.9 Hz), 3.93 (1H, qd, J = 7.2, 3.9 Hz), 3.70 (2H, s), 3.62 (1H, s), 3.39 (1H, d, J = 3.0 Hz), 3.01 (1H, s), 2.18 (1H, d, J = 9.3 Hz), 1.64–1.51 (6H, m), 1.51–1.44 (12H, m), 1.44–1.34 (4H, m); 13C-NMR δ (50 MHz, CDCl3): 175.73, 174.31, 173.44, 173.35, 156.61, 137.74, 137.35, 137.27, 129.10, 128.74, 127.96, 81.70, 64.54, 57.45, 56.93, 52.57, 51.13, 49.66, 48.72, 47.38, 37.86, 28.42, 27.83, 23.69, 17.46, 17.28. (+)ESI-MS (m/z): [M + Na]+ 624.4. Anal. calcd for C30H43N5O6S (601.3): C, 59.88; H, 7.20; N, 11.64; found C, 59.96; H, 7.23; N, 11.61. IR (KBr): ν = 3327, 2981, 1660, 1531, 1455 cm−1.
16b (white solid, Mp = 134 °C); yield 37%. αMeOHD = −66.1. 1H-NMR δ (200 MHz, CDCl3): 7.96 (1H, s), 7.43 (1H, d, J = 7.44 Hz), 7.27–7.26 (5H, m), 6.75 (1H, d, J = 3.6 Hz), 6.58–6.54 (1H, m), 6.28–6.24 (1H, m), 5.29 (1H, s), 4.88 (1H, s), 4.33 (1H, t, J = 7.3 Hz), 4.24 (1H, d, J = 3.6 Hz), 4.06–4.01 (1H, m), 3.60 (2H, s), 3.06 (1H, s), 3.03 (1H, s), 1.75 (1H, d, J = 9.5 Hz), 1.62–1.34 (22H, m). 13C-NMR δ (50 MHz, CDCl3): 176.38, 174.59, 173.81, 173.30, 157.38, 142.78, 138.37, 133.19, 128.77, 128.66, 127.42, 81.70, 70.50, 57.52, 54.27, 53.53, 52.67, 50.06, 49.96, 45.88, 39.25, 28.42, 27.71, 23.96, 17.72, 17.42. (+)ESI-MS (m/z): [M + Na]+ 624.4. Anal. calcd for C30H43N5O6S (601.3): C, 59.88; H, 7.20; N, 11.64; found C, 59.92; H, 7.25; N, 11.60. IR (KBr): ν = 3310, 2981, 1657, 1532 cm−1.
(1R,2R,3R,4S)-2-Amino-N-((S)-1-((1-(((S)-1-amino-1-oxopropan-2-yl)amino)-2-methyl-1-oxopropan-2-yl)amino)-1-oxopropan-2-yl)-3-(benzylthio)bicyclo[2.2.1]hept-5-ene-2-carboxamide (17a). Compound 16a was dissolved in water (0.1 M) in a sealed tube for microwaves reactor. The solution was irradiated by microwaves under magnetic stirring for 30 min at 150 °C. The reaction was monitored by Tlc (MeOH
:
DCM, 1
:
10; detected by ninhydrin). If not finished, another round of 20 minutes was performed. Upon consumption of the starting material water was evaporated at reduced pressure. The crude was purified by flash chromatography (GraceResolv silica cartridges; eluent MeOH
:
DCM, 1
:
20 to 1
:
1) to afford compound 17a in a pure form as white solid (88% yield). The reaction was performed with comparable yield from 0.1 g to 0.4 g. Mp 65 °C; αMeOHD = +38.57. 1H-NMR δ (200 MHz, CDCl3): 7.77 (1H, d, J = 3.6 Hz), 7.33–7.16 (7H, m), 6.52 (1H, s), 6.42 (1H, dd, J = 5.6, 3.0 Hz), 6.19 (1H, dd, J = 5.6, 3.0 Hz), 5.40 (1H, s), 4.42–4.35 (1H, m), 4.00 (1H, d, J = 3.4 Hz), 3.97–3.84 (1H, m), 3.69 (1H, d, J = 13.6 Hz, AB system), 3.64 (1H, d, J = 13.7 Hz, AB system), 3.11 (1H, s), 2.77 (1H, s), 2.04 (1H, d, J = 8.8 Hz), 1.53–1.34 (15H, m); 13C-NMR δ (50 MHz, CDCl3): 178.48, 175.69, 173.56, 173.03, 140.10, 139.48, 134.94, 128.96, 128.86, 127.35, 66.77, 56.96, 55.61, 54.81, 51.44, 49.87, 49.73, 45.72, 38.57, 27.49, 23.93, 17.54, 16.52. (+)ESI-MS (m/z): [M + Na]+ 524.4. Anal. calcd for C25H35N5O4S (501.3): C, 59.86; H, 7.03; N, 13.96; found C, 59.82; H, 7.09; N, 13.91; IR (KBr): ν = 3310, 2980, 1657, 1522 cm−1.
(1R,2S,3S,4S)-2-Amino-N-((S)-1-((1-(((S)-1-amino-1-oxopropan-2-yl)amino)-2-methyl-1-oxopropan-2-yl)amino)-1-oxopropan-2-yl)-3-(benzylthio)bicyclo[2.2.1]heptane-2-carboxamide (17b). Compound 16b was dissolved in water (0.1 M) in a sealed tube for microwaves reactor. The solution was irradiated by microwaves under magnetic stirring for 30 min at 150 °C. The reaction was monitored by Tlc (MeOH
:
DCM, 1
:
10; detected by ninhydrin). If not finished, another round of 20 minutes was performed. Upon consumption of the starting material water was evaporated at reduced pressure. The crude of reaction was purified by chromatography (GraceResolv silica cartridges, eluent MeOH
:
DCM, 1
:
20 to 1
:
15) to afford compound 17b in a pure form as colorless wax (82% yield). The reaction was performed with comparable yield from 0.1 g to 0.4 g, αMeOHD = −7.65. 1H-NMR δ (200 MHz, CDCl3): 8.10 (1H, d, J = 4.6 Hz), 7.36–7.20 (5H, m), 7.10 (1H, d, J = 7.7 Hz), 7.02 (1H, s), 6.71 (1H, s), 6.43 (1H, dd, J = 5.5, 2.9 Hz), 6.24 (1H, dd, J = 5.6, 3.0 Hz), 5.49 (1H, s), 4.46–4.23 (1H, m), 4.12 (1H, dt, J = 12.0, 7.1 Hz), 3.92 (1H, d, J 3.3 Hz), 3.70 (2H, s), 3.01 (1H, s), 2.95 (1H, s), 2.00 (1H, d, J = 9.5 Hz), 1.67–1.25 (15H, m); 13C-NMR δ (50 MHz, CDCl3): 178.14, 175.44, 173.72, 172.79, 140.13, 138.2, 134.98, 128.91, 128.79, 127.55, 66.53, 57.15, 55.01, 54.31, 51.45, 49.71, 48.58, 46.25, 37.38, 26.78, 24.58, 17.50, 16.9. (+)ESI-MS (m/z): [M + Na]+ 524.4. Anal. calcd for C25H35N5O4S (501.3): C, 59.86; H, 7.03; N, 13.96; found C, 59.89; H, 7.09; N, 13.89. IR (KBr): ν = 3317, 2975, 1653, 1521 cm−1.
(1R,2R,3R,4S)-2-((S)-2-Acetamidopropanamido)-N-((S)-1-((1-(((S)-1-amino-1-oxopropan-2-yl)amino)-2-methyl-1-oxopropan-2-yl)amino)-1-oxopropan-2-yl)-3-(benzylthio)bicyclo[2.2.1]hept-5-ene-2-carboxamide (2a). (l)Ac-Ala-OH (1 eq.) was dissolved in dry DCM (0.1 M) and HOAt (1.1 eq.) and EDC (1.1 eq.) were added under nitrogen at 0 °C. The mixture was made to react under stirring at 0 °C for 1 h. After that, 17a (NRB-Ala-Aib-AlaCONH2) (1.1 eq.) and DIPEA (2.2 eq.) were added followed by additional DIPEA to reach pH = 8, and then the reaction mixture was let to react at room temperature for 12 h. The reaction was monitored by HPTlc (MeOH
:
DCM, 1
:
10; detected by ninhydrin or UV light and checked by 1H-NMR). Upon the consumption of the starting material the reaction mixture was washed with saturated NH4Cl, saturated NaHCO3, and brine. The NH4Cl, saturated solution was extracted 3 times with EtOAc. The combined organic phases were dried over Na2SO4 and the solvent evaporated at reduced pressure. The crude was purified by inverse phase HPLC (eluent 95% H2O, 5% CH3CN, 0.1% TFA to 70% H2O 30% CH3CN 0.1% TFA) to afford pure compound 2a as solid wax (40% yield). Recovered starting material (40%). The reaction was performed with comparable yield from 0.1 g to 2 g. αMeOHD = 146.4. 1H-NMR δ (500 MHz, CD3CN): 7.96 (1H, bs), 7.85 (1H, s), 7.65 (1H, bs), 7.41–7.28 (7H, m), 7.10 (1H, s), 6.28 (1H, dd, J = 5.6, 2.8 Hz), 6.19 (1H, bs), 6.09 (1H, s), 4.19–3.94 (1H, m), 4.15–4.07 (1H, m), 4.05–4.00 (1H, m), 3.83 (1H, d, J = 13.5 Hz, AB system), 3.66 (1H, d, J = 13.5 Hz, AB system), 3.64 (1H, s), 3.36 (1H, s), 2.90 (1H, s), 2.20 (1H, d, J = 8.2 Hz), 2.04 (3H, s), 1.51 (3H, s), 1.50 (3H, s), 1.48–1.45 (4H, m), 1.39 (3H, d, J = 3.9 Hz), 1.37 (3H, d, J = 3.9 Hz); 13C-NMR δ (126 MHz, CD3CN): 176.42, 175.37, 174.63, 174.59, 173.32, 173.13, 137.81, 137.35, 136.48, 131.26, 128.77, 127.46, 67.76, 56.68, 56.43, 52.39, 52.26, 50.48, 49.70, 48.39, 47.16, 36.32, 26.61, 22.70, 21.92, 16.54, 16.03, 15.80. (+)ESI-MS (m/z): [M + Na]+ 637.4. Anal. calcd for C30H42N6O6S (614.3): C, 58.61; H, 6.89; N, 13.67; found C, 58.62; H, 6.89; N, 13.68. IR (KBr): ν = 3333, 2974, 1656, 1529 cm−1.
(1S,2S,3S,4R)-2-((S)-2-Acetamidopropanamido)-N-((S)-1-((1-(((S)-1-amino-1-oxopropan-2-yl)amino)-2-methyl-1-oxopropan-2-yl)amino)-1-oxopropan-2-yl)-3-(benzylthio)bicyclo[2.2.1]hept-5-ene-2-carboxamide (2b). In a sealed tube for microwaves reactor, compound 17b was dissolved in THF (0.1 M) and EEDQ (1.1 eq.) and (l)-Ac-Ala-OH (1 eq.) were added. The solution was irradiated by microwave under magnetic stirring for 30 min at 60 °C (power: 80 Watt) using the air compressing cooling system of the reactor to keep down the temperature. The reaction was monitored by HPTlC (5 × 7.5 cm silica gel 60 F254, MERCK) (MeOH
:
DCM, 1
:
10; detected by ninhydrin). If not finished another round of 30 minutes was performed. Upon consumption of the starting material the tube was cooled down in an ice bath and the precipitate was filtered affording pure compound 2b as white solid (Mp: 76 °C) without the need of further purification (yield: 60%). The slow precipitation in a concentrated solution of compound 2b in CH3CN permitted the obtainment of a single crystal for X-ray analysis. The reaction was performed with comparable yield from 50 mg to 100 mg, αMeOHD = −89.6. 1H-NMR δ (500 MHz, CD3CN): 7.73 (1H, s), 7.52 (1H, d, J = 4.8 Hz), 7.35–7.31 (5H, m), 7.29–7.24 (1H, m), 7.08 (1H, s), 7.02 (1H, s), 6.95 (1H, d, J = 5.8 Hz), 6.41 (1H, dd, J = 5.6, 2.9 Hz), 6.29 (1H, dd, J = 5.7, 2.9 Hz), 5.58 (1H, s), 4.28 (1H, dq, J = 5.8, 7.15 Hz), 4.16 (1H, d, J = 3.5 Hz), 4.07 (1H, dq, J = 7.36, 7.36 Hz), 3.99 (1H, dq, J = 4.8, 7.3 Hz), 3.81 (1H, d, J = 12.6 Hz, AB system), 3.77 (1H, d, J = 12.6 Hz, AB system), 3.33 (1H, s), 3.04 (1H, s), 1.95 (3H, s), 1.80 (1H, d, J = 9.3 Hz), 1.49 (6H, d, J = 1.3 Hz), 1.45–1.38 (4H, m), 1.34 (3H, d, J = 5.0 Hz), 1.33 (3H, d, J = 5.2 Hz); 13C-NMR δ (126 MHz, CD3CN): 175.46, 174.49, 174.16, 173.93, 173.90, 171.45, 139.75, 138.60, 134.81, 128.78, 128.47, 126.96, 68.17, 56.60, 55.06, 52.40, 51.99, 50.31, 49.67, 49.08, 45.45, 38.10, 26.26, 23.19, 21.93, 16.95, 16.11, 15.83. (+)ESI-MS (m/z): [M + Na]+ 637.4. Anal. calcd for C30H42N6O6S (614.3): C, 58.61; H, 6.89; N, 13.67; found C, 58.66; H, 6.93; N, 13.64. IR (KBr): ν = 3310, 2984, 1656, 1533 cm−1.
Acknowledgements
This work was partially supported by Ministero dell'Università e della Ricerca (Prin 2010 “Synthesis and biomedical applications of tumor-targeting peptidomimetics” prot. 2010NRREPL. We also acknowledge the CINECA and the Regione Lombardia award under the LISA initiative, for the availability of high performance computing resources and support.
References
- A. Mullard, Nat. Rev. Drug Discovery, 2012, 11, 173–175 CrossRef CAS PubMed.
- R. J. Bienstock, Curr. Pharm. Des., 2012, 18, 1240–1254 CrossRef CAS.
- M. Rubinstein and M. Y. Niv, Biopolymers, 2009, 91, 505–513 CrossRef CAS PubMed; V. M. Ahrens, K. Bellmann-Sickert and A. G. Beck-Sickinger, Future Med. Chem., 2012, 4, 1567–1586 CrossRef PubMed.
- L. Diao and B. Meibohm, Clin. Pharmacokinet., 2013, 52, 855–868 CrossRef CAS PubMed.
-
(a) I. L. Karle and P. Balaram, Biochemistry, 1990, 29, 6747–6756 CrossRef CAS;
(b) C. Toniolo, M. Crisma, F. Formaggio and C. Peggion, Biopolymers, 2001, 60, 396–419 CrossRef CAS;
(c) M. C. Venkatachalam, Biopolymers, 1968, 6, 1425–1436 CrossRef PubMed;
(d) C. Toniolo, Crit. Rev. Biochem., 1980, 9, 1–44 CrossRef CAS;
(e) G. D. Rose, L. M. Gierasch and J. A. Smith, Adv. Protein Chem., 1985, 37, 1–109 CrossRef CAS;
(f) G. Némethy and M. P. Printz, Macromolecules, 1972, 5, 755–758 CrossRef;
(g) B. W. Matthews, Macromolecules, 1972, 5, 818–819 CrossRef CAS;
(h) C. Toniolo and E. Benedetti, Trends Biochem. Sci., 1991, 16, 350–353 CrossRef CAS;
(i) C. Toniolo, M. Crisma, F. Formaggio, C. Peggion, Q. B. Broxterman and B. Kaptein, Biopolymers, 2004, 76, 162–176 CrossRef CAS PubMed.
-
(a) L. Gentilucci, D. R. Marco and L. Cerisoli, Curr. Pharm. Des., 2010, 16, 3185–3203 CrossRef CAS;
(b) S. Pellegrino, A. Contini, F. Clerici, A. Gori, D. Nava and M. L. Gelmi, Chem.–Eur. J., 2012, 18, 8705 CrossRef PubMed;
(c) S. Pellegrino, A. Contini, M. L. Gelmi, L. Lo Presti, R. Soave and E. Erba, J. Org. Chem., 2014, 79, 3094 CrossRef CAS PubMed.
- E. Longo, A. Moretto, F. Formaggio and C. Toniolo, Chirality, 2011, 23, 756–760 CrossRef CAS.
- F. Caputo, F. Clerici, M. L. Gelmi, S. Pellegrino and D. Pocar, Tetrahedron: Asymmetry, 2006, 17, 1430–1436 CrossRef CAS PubMed.
- S. Pellegrino, F. Clerici and M. L. Gelmi, Tetrahedron, 2008, 64, 5657–5665 CrossRef CAS PubMed.
- A. Ruffian, A. Casoni, S. Pellegrino, M. L. Gelmi, R. Soave and F. Clerici, Tetrahedron, 2012, 68, 1951–1962 CrossRef PubMed.
- M. L. Gelmi, C. Cattaneo, S. Pellegrino, F. Clerici, M. Montali and C. Martini, J. Org. Chem., 2007, 72, 9811–9814 CrossRef CAS PubMed.
-
(a) M. A. Sussman, S. Welch, A. Walker, R. Klevitsky, T. E. Hewett, R. L. Price, E. Schaefer and K. Yager, J. Clin. Invest., 2000, 105, 875–886 CrossRef CAS PubMed;
(b) M. Satoh, H. Ogita, K. Takeshita, Y. Mukai, D. J. Kwiatkowski and J. K. Liao, Proc. Natl. Acad. Sci. U. S. A., 2006, 103, 7432–7437 CrossRef CAS PubMed.
- A. Ruffian, N. Ferri, S. K. Bernini, C. Ricci, A. Corsini, I. Maffucci, F. Clerici and A. Contini, J. Med. Chem., 2014, 57, 2953–2962 CrossRef PubMed.
-
(a) N. Ferri, S. K. Bernini, A. Corsini, F. Clerici, E. Erba, S. Stragliotto and A. Contini, Med. Chem. Commun., 2013, 4, 537–541 RSC;
(b) N. Ferri, A. Corsini, P. Bottino, F. Clerici and A. Contini, J. Med. Chem., 2009, 52, 4087–4090 CrossRef CAS PubMed.
- A. Contini, unpublished results. On the Rac1 side, interactions with Tiam1 are mediated by two loops, switch 1 (AAs 25–39) and switch 2 (AAs 57–75), and by non-conserved residues between switches, ensuring proper GEF/Rac1 pairing. (Worthylake, D. K.; Rossman, K. L.; Sondek, J., Nature, 2000, 408, 682.) Preliminary results from our laboratory show hot and warm spots in the 13 residue long helix of Tiam1, CR3 (I1187-L1199); however, this portion cannot be used as a standalone peptide inhibitor, since is predicted to be disordered. However, we observed trough preliminary calculations that non-natural helical peptides mimicking the Tiam1 CR3 sequence could maintain the ability to bind Rac1, without altering the conformation of switch 1 and 2.
- I. Maffucci, S. Pellegrino, J. Clayden and A. Contini, J. Phys. Chem. B, 2015, 119, 1350–1361 CrossRef CAS PubMed.
- A. Carpino, D. Sadat-Aalaee, H. G. Chao and R. H. De Selms, J. Am. Chem. Soc., 1990, 112, 9651–9652 CrossRef.
- A. Thaqi, A. McCluskey and J. L. Scott, Tetrahedron Lett., 2008, 49, 6962–6964 CrossRef CAS PubMed.
- A. Mahindra, K. Nooney, S. Uraon, K. K. Sharma and R. Jain, RCS Adv., 2013, 3, 16810–16816 CAS.
- V. Santagada, F. Fiorino, E. Perissutti, B. Severino, V. De Filippis, B. Vivenzio and G. Caliendo, Tetrahedron Lett., 2001, 42, 5171–5173 CrossRef CAS.
- C. Toniolo, E. Valente, F. Formaggio, M. Crisma, G. Pilloni, C. Corvaja, A. Toffoletti, G. V. Martinez, M. P. Hanson, G. L. Millhauser, C. George and J. L. Flippen-Anderson, J. Pept. Sci., 1995, 1, 45–57 CrossRef CAS PubMed.
- G. R. Desiraju, Chem. Commun., 2005, 28, 2995–3001 RSC.
- E. Borsini, G. Broggini, A. Contini and G. Zecchi, Eur. J. Org. Chem., 2008, 2808–2816 CrossRef CAS.
- A. Contini, D. Nava and P. Trimarco, J. Org. Chem., 2006, 71, 159–166 CrossRef CAS PubMed.
- A. Contini, S. Leone, S. Menichetti, C. Viglianisi and P. Trimarco, J. Org. Chem., 2006, 71, 5507–5514 CrossRef CAS PubMed.
- L. Lo Presti, R. Soave and R. Destro, J. Phys. Chem. B, 2006, 110, 6405–6414 CrossRef CAS PubMed.
- M. C. Aversa, C. Barattucci, P. Bonaccorsi and A. Contini, J. Phys. Org. Chem., 2009, 22, 1048–1057 CrossRef CAS.
- A. Contini and E. Erba, RSC Adv., 2012, 2, 10652–10660 RSC.
- K. Wuthrich, in NMR of Proteins and Nucleic Acids, Wiley, NewYork, NY, 1986 Search PubMed.
- G. Jung, H. Bruckner, R. Bosch, V. Winter, H. Schaal and J. Strahle, Liebigs Ann. Chem., 1983, 7, 1096–1106 CrossRef.
- T. A. Darden D. A. Case, T. E. Cheatham III, C. L. Simmerling, J. Wang, R. E. Duke, R. Luo, R. C. Walker, W. Zhang, K. M. Merz, B. Roberts, S. Hayik, A. Roitberg, G. Seabra, J. Swails, A. W. Goetz, I. Kolossváry, K. F. Wong, F. Paesani, J. Vanicek, R. M. Wolf, J. Liu, X. Wu, S. R. Brozell, T. Steinbrecher, H. Gohlke, Q. Cai, X. Ye, J. Wang, M.-J. Hsieh, G. Cui, D. R. Roe, D. H. Mathews, M. G. Seetin, R. Salomon-Ferrer, C. Sagui, V. Babin, T. Luchko, S. Gusarov, A. Kovalenko and P. A. Kollman, AMBER 12, 2012 Search PubMed.
- Y. Sugita and Y. Okamoto, Chem. Phys. Lett., 1999, 314, 141–151 CrossRef CAS.
- R. P. Joosten, T. A. H. te Beek, E. Krieger, M. L. Hekkelman, R. W. W. Hooft, R. Schneider, C. Sander and G. Vriend, Nucleic Acids Res., 2011, 39, D411–D419 CrossRef CAS PubMed.
Footnote |
† Electronic supplementary information (ESI) available: Experimental protocols for the synthesis of peptides 2a and 2b 1H and 13C spectra for all compounds and crystallographic data in CIF. CCDC 989313 and 989314. For ESI and crystallographic data in CIF or other electronic format see DOI: 10.1039/c5ra03805g |
|
This journal is © The Royal Society of Chemistry 2015 |
Click here to see how this site uses Cookies. View our privacy policy here.