DOI:
10.1039/C4RA16587J
(Paper)
RSC Adv., 2015,
5, 21909-21915
The simultaneous determination of omethoate and dichlorvos pesticides in grain samples using a palladium and graphene composite modified glassy carbon electrode
Received
17th December 2014
, Accepted 13th February 2015
First published on 13th February 2015
Abstract
An electrochemical sensor based on a palladium (Pd) and graphene (Gr) composite modified glassy carbon electrode (GCE) was fabricated, characterised and used for the simultaneous determination of omethoate (OMT) and dichlorvos (DCV) in grain samples. The Pd/Gr/GCE was characterized by scanning electron microscopy (SEM) and cyclic voltammograms (CV). The modified electrode displayed a greatly improved voltammetric response to OMT and DCV. Square wave voltammetry (SWV) was used for the individual and simultaneous determination of OMT and DCV. Various conditions were optimized, such as the pH of the buffer, the applied sample volume, the accumulation potential, the accumulation time, the square wave frequency and the step potential. Well defined reduction (OMT at C
O, DCV at C
C) peaks were obtained over the potential maximum in an acidic medium in phosphate buffer solution at −0.50 V and −0.80 V for both OMT and DCV. Low current peaks were obtained over the concentration of 3.50 × 10−8 M with detection limits 2.47 × 10−10 M and 3.28 × 10−10 M for OMT and DCV respectively. The composite modified electrode showed good stability and reproducibility. Therefore, the proposed method was successfully applied as an economical and simple sensor with a shorter analysis time.
1. Introduction
Nanostructured materials have received a great deal of attention due to their unique chemical and physical properties, depending upon their size and shape.1 Graphene (Gr), a two dimensional carbon nanomaterial with outstanding physical and chemical properties, has recently become one of the most exciting topics of both fundamental science and applied research.2 As a counterpart of graphite, with well separated 2D aromatic sheets, Gr possesses sp2 conjugated bonds in the carbon lattice and has remarkably high electron mobilities under ambient conditions.3 Moreover, Gr has a very large specific surface area with a low manufacturing cost and excellent mechanical strength.4
Even though palladium (Pd) itself has been proven to be a suitable catalyst for the electrooxidation of alcohols in alkaline media, more efforts are needed for the further improvement of the electrocatalytic performance of Pd-based catalysts. Supporting the catalysts on materials with large surface areas, such as carbon black, activated carbon, carbon nanofibers and carbon nanotubes, is one approach.5–7 Palladium nanostructures are of great interest due to their invaluable and excellent catalytic performance and for possessing properties such as lower cost, high efficiency and selectivity (Fig. 1).8–12 A large number of carbon–carbon bond forming reactions in organic chemistry, such as Suzuki, Heck, Stille coupling, hydrogenation/dehydrogenation reactions, low temperature reduction of automobile pollutants and petroleum cracking, depend on catalysts which are based upon Pd and its compounds.13–16 A combination of Pd nanostructures and carbon materials was found to enhance the stability and activity of catalysts, including carbon nanotubes, nanorods and the new emerging graphene.17,18 Muniyandi Rajkumar et al. reported the preparation of single step electrochemical fabrication of highly loaded palladium nanoparticles decorated on chemically reduced graphene oxide and the electrocatalytic applications.19 Qiyu Wang et al. reported the synthesis of flower-shape palladium nanostructures on graphene oxide for electrocatalytic applications.20 A number of scientists previously reported the preparation of palladium and graphene composite modified glassy carbon electrodes for use in electrochemical applications.21–25
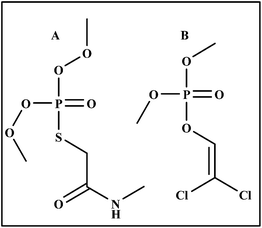 |
| Fig. 1 Molecular structure of OMT (A) and DCV (B). | |
The detection of organophosphorous compounds is of great practical importance and has received escalating interest. Omethoate (OMT) and dichlorvos (DCV) are extensively used organophosphorous pesticides in India. They efficiently kill insects in agricultural field crops. A large number of papers have been published on the determination of omethoate by mass spectrometry-gas chromatography and voltammetry.26–35 Djenaine De Souza et al. reported on using an electroanalytical method for the determination of DCV using gold-disk microelectrodes.36 Yanhong Bai et al. reported the reduction of DCV and OMT residues by an O2 plasma treatment.37 Yugui Tao et al. reported on the simultaneous determination of OMT and DCV by capillary electrophoresis.38 Nevertheless, to the best of our knowledge, there is no electroanalytical report concerning the simultaneous determination of OMT and DCV using a palladium and graphene nanocomposite modified glassy carbon electrode.
Due to the advantages of Gr and Pd nanoparticles and their synergistic effects, a Pd/Gr nanocomposite modified electrode was prepared and found to exhibit better electrochemical performances. The voltammetric sensor developed was successfully applied to the simultaneous determination of OMT and DCV with good electrocatalytic activity, high sensitivity, good repeatability, long term stability and low cost.
2. Experimental
2.1. Apparatus
Electrochemical studies were carried out by an Autolab PG STAT101 supplied by Metrohm Autolab B.V. Netherlands. A three electrode system comprising a glassy carbon electrode modified with palladium (Pd) and graphene (Gr) composites served as a working electrode. Saturated Ag/AgCl/KCl was used as a reference electrode and Pt wire as a counter electrode. The electrode surface morphology study was carried out using scanning electron microscopy (SEM), instrument model OXFORD INCA PENTA FETX3 CARL ZEISS from Japan. An Elico LI-120 pH meter, supplied by Elico Ltd, Hyderabad, India, was used to determine the pH of the buffer solution.
2.2. Chemicals and reagents
OMT and DCV were purchased from Siddarth. Inc. Hyderabad, India and their stock solutions were prepared in methanol. Phosphate buffer (PB) solutions at different pH values were prepared by mixing standard solutions of 0.1 M NaH2PO4 and 0.1 M Na2HPO4 and adjusting the pH with 0.1 M H3PO4 or 0.1 M NaOH. All solutions were filtered through a 0.45 μm membrane filter and then degassed by sonication and evacuation. All chemicals were of analytical reagent grade and all the aqueous solutions were prepared with redistilled deionised water. These solutions were stored in the dark at 4 °C.
2.3. Sample preparation of OMT and DCV in grain samples
Known amounts of the standard solutions of OMT and DCV were added to a 25 mL aliquot of grain samples (green peas and field bean from Tirupati, India) giving a final concentration of 10 μL of OMT and DCV. Each sample was mixed with 10.0 mL of 0.20 mol L−1 PB solution (pH 5.0). No further sample treatment was done. The OMT and DCV concentrations were determined by three successive additions of aliquots of the standard OMT and DCV solutions.
2.4. Fabrication of the Pd/Gr/GCE
Prior to the electrodeposition process, the bare glassy carbon electrode was initially polished with 0.05 μM alumina powder using a BAS polishing kit and ultrasonically cleaned in water for 1 min. The electrode was then washed with double distilled water and used for electrodeposition. The Pd/Gr nanocomposite was fabricated on the GCE surface by a simple two-step process. According to our previous paper, the prepared Gr solution was drop casted on the pre-cleaned GCE and dried in an air oven at 30 °C.39 Then the Gr modified GCE was shifted to an electrochemical cell with 10 mL of 0.5 M H2SO4 containing 1 mM H2PdCl4. Consecutive cyclic voltammograms were recorded in the potential range of 1.0 V to −1.0 V vs. a Ag/AgCl/KCl reference electrode at a scan rate of 100 mV s−1 in 100 s deposition time to obtain stable voltammograms. Well defined redox voltammograms were obtained at potentials of −0.25 V and 0.25 V in 0.5 M H2SO4 solution. The resulting modified electrode, denoted as a Pd/Gr nanocomposite film modified GCE, was then rinsed with double distilled water and used for the electrochemical studies. The electrode modification process and consecutive cyclic voltammograms are shown in Fig. 2A and B.
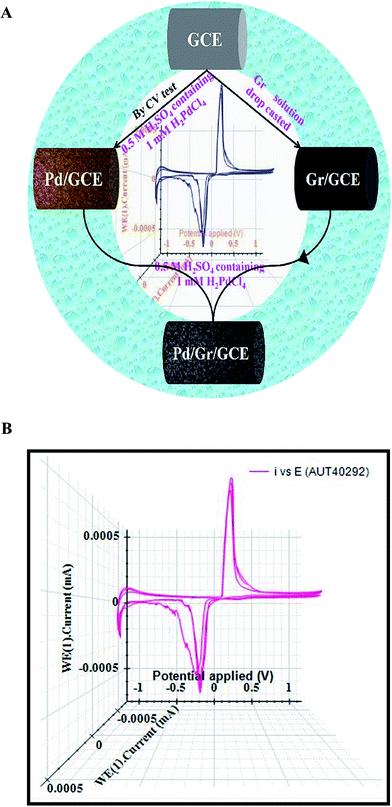 |
| Fig. 2 (A) Schematic graphical representation of the fabrication of palladium and graphene nanocomposites on the surface of the glassy carbon electrode. (B) Electrodeposition of Pd nanoparticles on Gr/GCE from 0.5 M H2SO4 containing 1.0 mM H2PdCl4, potential scan between −1.0 V to 1.0 V for three consecutive cyclic voltammograms at the scan rate of 100 mV s−1. | |
3. Results and discussions
3.1. Characterisation of the Pd/Gr/GCE
The cyclic voltammograms (CV) show the electrode deposition process of Pd nanoparticles on Gr/GCE surface, which begins at the positive potential of 1.0 V and ends at the negative potential of −1.0 V, as shown in Fig. 3. The oxidation peak of the Pd particles occurs at a potential of 0.25 V and the reduction process takes place at −0.25 V. The fabricated Pd particles were oxidized to form a Pd oxide layer at 0.25 V on the electrode surface. After that, the existing Pd oxide was reduced during the negative scans because of the hydrogen adsorption process. Throughout this repetitive cycling process, the reduction and oxidation peaks of the Pd particles were clearly growing on the Gr/GCE surface. It is clear that the addition of Pd nanoparticles onto the surface of Gr/GCE. Pd/Gr was made with all volumes of H2PdCl4, from 1 mg to 5 mg, and the voltammetric response with regards to the stability, at each volume, was tested by use of a CV in 0.5 M H2SO4 solution over a potential range of 1.0 V to −1.0 V in 100 s (Fig. 3). The CV revealed that as the amount of H2PdCl4 increased from 2.5 mg to 4.5 mg, the redox peak current enhanced linearly and the Pd nanoparticles were tightly bound on the surface of Gr/GCE. Once the amount of H2PdCl4 is raised above 5 mg, the current response drops off, therefore the surface of Gr/GCE becomes unstable due to Pd nanoparticles being overloaded on the Gr/GCE. This is clearly shown in Fig. 3 from cycle ‘c’ to cycle ‘a’. Therefore the preferred amount for fabricating Pd nanoparticles on the surface of the Gr/GCE by cyclic voltammetry for electroanalytical applications is between 2.5 mg to 4.5 mg of H2PdCl4.
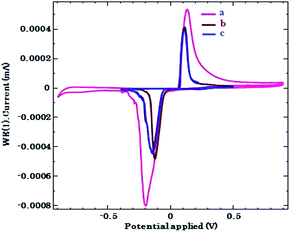 |
| Fig. 3 Cyclic voltammetric behaviour of Pd nanoparticles on the Gr/GCE, different Pd wt%: 15 wt% (a), 25 wt% (b) and 35 wt% (c) in 0.5 M H2SO4 solution at a scan rate of 100 mV s−1. | |
3.2. Morphological study
The morphology and size of the Pd nanoparticles and the Pd/Gr/GCE were examined by SEM analysis. Fig. 4A shows the formation of Pd nanoparticles across the GCE surface. Here, we can clearly see that the formed Pd nanoparticles were equally scattered on the surface of the GCE. Fig. 4B shows the SEM images of the Pd/Gr/GCE which clearly indicates that the Pd nanoparticles are binding up homogenously across the Gr/GCE and that the distribution of the nanoparticles is almost uniform on the electrode surface. The immobilized Pd/Gr nanocomposites on the surface of the GCE remain stable and there is no deformation in shape. Finally, from these results it is evident from the size and morphology that the Pd nanoparticles were fully attached to the Gr/GCE surface.
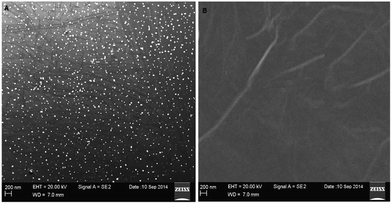 |
| Fig. 4 SEM images of (A) Pd nanoparticles on GCE surface and (B), Pd/Gr/GCE. | |
3.3. The simultaneous determination of OMT and DCV
The Pd/Gr nanocomposite modified GCE could be directly employed for the simultaneous determination of OMT and DCV. In Fig. 5, the square wave voltammetry curve ‘a’ represents the GCE response, curve ‘b’ represents the Pd/GCE nanocomposite film response, curve ‘c’ represents the Gr/GCE response and curve ‘d’ represents the Pd/Gr/GCE response for the simultaneous determination of OMT and DCV. Here we also compare the individual electrocatalytic reduction behaviour of OMT and DCV at the Pd/Gr/GCE. Comparing the Pd/Gr nanocomposite film with the Pd nanoparticles, the Pd/Gr nanocomposite shows well defined and obvious electrocatalytic peaks for the determination of OMT and DCV. At the same time, the Pd nanoparticle modified electrode also gives two separate peaks, but the peaks were not obvious, like the response found using Pd/Gr nanocomposite film. In this, OMT reduction takes place at around −0.45 V and DCV around at −0.77 V. Comparing these results, we can see that the Pd/Gr nanocomposite film modification is more suitable for the simultaneous detection of OMT and DCV than the other electrodes. This may be due to the presence of the Pd nanoparticles, which act as electroactive centres for the determination of these compounds. In addition, the presence of Gr enhances the peak current and the potential shift supports the simultaneous determination of OMT and DCV. Subsequently, based on this framework, we conclude that the combination of Pd/Gr nanocomposite film is more suitable for these type of analyses.19,21
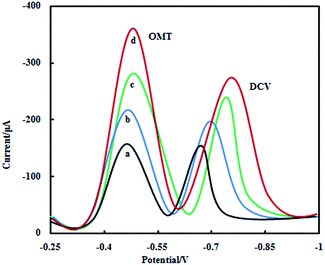 |
| Fig. 5 SWV obtained under optimised parameters in 0.2 mol L−1 PB solution (pH 5.0) for OMT and DCV determination on (a) the GCE, (b) the Pd/GCE, (c) the Gr/GCE and (d) the Pd/Gr/GCE at 3.5 × 10−8 M. | |
3.4. Effect of the accumulation potential and time
The influence of the accumulation potential on the SWV signal was determined with an accumulation time of 60 s at the Pd/Gr/GCE for 3.5 × 10−8 M of OMT and DCV. It was observed that the Ip value was at −0.45 V for a pH of 5.0 due to an increased accumulation rate because of the more positive alignment of molecules at the electrode solution interface by the electric field. For the surface adsorption of pesticides, the time effect of the surface accumulation was investigated at potentials from −0.2 V to −1.00 V. It was found that the peak current of the pesticide was not very dependent on the initial potential setting. However, it quickly improved with extending the time of accumulation in the first 60 s and then became constant afterward. The optimized accumulation time was selected as 60 s.
3.5. Effect of pH
The effect of solution pH on the response of OMT and DCV on the Pd/Gr modified GCE was investigated at 3.5 × 10−8 M, as shown in Fig. 6. The cathodic peak currents of OMT and DCV increased slowly with increasing pH from 2.0 to 5.0 (Fig. 6). At a pH higher than 5.0, the peak currents decreased quickly with increasing pH from 5.0 to 10. Hence, the peak current becomes larger with the pH increasing from 3.0 to 5.0. However, at a pH over 5.0, OMT and DCV may be become protonated as cations and they can start to be reduced. Also, the carbonyl containing functional groups in DCV may become protonated at the Pd/Gr modified electrode and possess negative charges at a pH of 5.0. Therefore, electrostatic repulsion between the analytes and the electrode may be one of the reasons for the decrease of the peak currents of OMT and DCV when the pH increases from 5.0 to 10.0. The suggested electrode reduction process involves two proton and two electron transfers.40 In order to achieve high sensitivity, a pH 5.0 was chosen for the simultaneous detection of OMT and DCV.
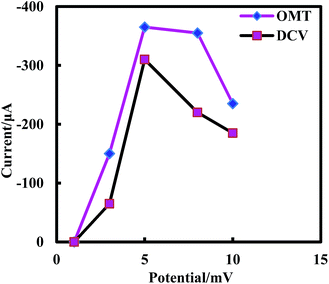 |
| Fig. 6 Effect of the pH on the peak current of OMT and DCV at the Pd/Gr/GCE in PB solution at 3.5 × 10−8 M. | |
3.6. Analytical response of OMT and DCV
By means of the SWV optimised conditions, as previously described, the proposed Pd/Gr/GCE was applied to investigate the electrochemical response as a function of the individual OMT and DCV concentrations. All measurements were made in triplicate. The analytical response shown in Fig. 7, has a linear response in the range of 3.5 × 10−8 M to 1.25 × 10−5 M for both OMT and DCV. The results of these experiments are shown individually in Fig. 8A and B. When the concentration of OMT and DCV were increased from 3.5 × 10−8 M to 1.25 × 10−5 M, the reduction peak current increased linearly. The linear regression equation for this region was: Y = −60.857x + 4.667, Y = −64.571x + 1 with correlation coefficients of R2 = 0.9975, 0.9939 and the limits of detection (S/N = 3) are 2.47 × 10−10 M and 3.28 × 10−10 M for OMT and DCV respectively. Thus, the Pd/Gr/GCE has a good electrocatalytic activity and adsorption capacity towards high concentrations OMT and DCV.
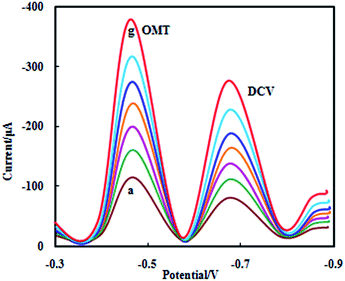 |
| Fig. 7 Simultaneous SWV responses of OMT and DCV at the Pd/Gr/GCE in PB solution (pH 5.0) with increasing concentrations (a–g) 3.5 × 10−8 M to 1.25 × 10−5 M. | |
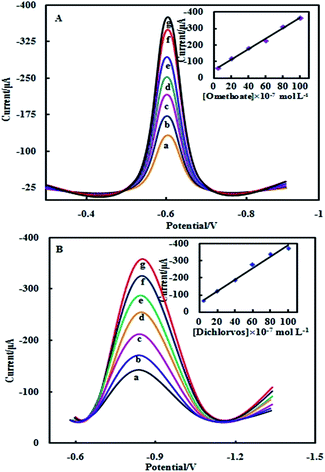 |
| Fig. 8 SWV responses of OMT (A) and DCV (B) at the Pd/Gr/GCE in PB solution (pH 5.0) with increasing concentrations (a–g) 3.5 × 10−8 M to 1.25 × 10−5 M. | |
3.7. Pre-treatment of real samples
Fresh grain samples (mean weight 5 g) were pulverised by a blender and placed in a 250 mL volumetric flask. As the concentration of pesticides in the grain samples were too low for direct detection, 2.0 mL of each of the standard solutions of the pesticides were sampled by pipetting. This aliquot was transferred to a flask containing 50.0 mL ethanol and 50 g anhydrous sodium sulphate was added to remove any water. The addition of 0.5 g activated charcoal then facilitated the removal of the interfering colouring matter. The mixture was shaken for 30 min and then the organophosphorous pesticides in the grain samples were extracted into the methanol phase. The sample was filtered, 25.0 mL of the methanol solution was transferred into an evaporating dish and reduced to 5 ml by flowing nitrogen gas into the cell. This aliquot was diluted to 10 mL with distilled water.
3.8. Determination of the pesticide in grain samples
The common grains of green peas and field bean were chosen for analysis. Each sample of the grains were treated as described above and 0.1 mL of the extract was transferred to the electrochemical cell for analysis. This allowed for calibration when analysing the grain extracts. The results (Table 1) showed that values of the pesticides found in the grain samples were in the range of 3.5 × 10−8 M to 1.25 × 10−5 M and the procedure was further validated by standard addition of the given pesticides. This showed good recovery values in the range of 96.00–99.71%.
Table 1 Recovery study of OMT and DCV in grain samples
Samples |
Added (M) |
Found (M) |
aRecovery (%) |
RSD |
Average of five determinations. |
Green peas |
1.25 × 10−5 |
1.23 × 10−5 |
98.40 |
1.75 |
2.25 × 10−6 |
2.23 × 10−6 |
99.11 |
2.05 |
3.50 × 10−7 |
3.48 × 10−7 |
99.42 |
2.15 |
3.50 × 10−8 |
3.49 × 10−8 |
99.71 |
2.24 |
Field bean |
1.25 × 10−5 |
1.20 × 10−5 |
96.00 |
0.78 |
2.25 × 10−6 |
2.21 × 10−6 |
98.22 |
1.63 |
3.50 × 10−7 |
3.47 × 10−7 |
99.14 |
2.10 |
3.50 × 10−8 |
3.48 × 10−8 |
99.42 |
2.15 |
3.9. Calibration curves and limits of detection
The above sample procedure was carried out for the individual pesticides. Calibration plots based on the dependence of the peak current on the concentration were linear. In addition to the calibration parameters, the precision for determining these compounds was also established by analysing five known solutions containing the lowest concentration on the calibration graph. The relative standard deviation (RSD) values obtained were 0.78 to 2.24% for OMT and DCV respectively. The detection limit values were found to be 2.47 × 10−10 M and 3.28 × 10−10 M for OMT and DCV respectively, which compare well with the detection limits obtained previously.36–38 Thus, these results clearly indicate that the proposed electrochemical method of analysis is reliable for the determination of the individual pesticides (Fig. 8). However, it should be noted that the linear calibration relationships were observed only at the actual peak potential. These changes showed small progressive peak potential shifts to more negative values with increases in concentration.
3.9.1. Reproducibility and stability. The stability of the Pd/Gr/GCE was investigated by CV (figure not shown). The Pd/Gr/GCE exhibits good measurement stability over 10 consecutive cycles of the CV test. A small decrease in peak current was observed with increases in the scanning cycle but the reduction peak current retained 99% of its initial current after it was tested by a consecutive CV scan. This indicates that the Pd/Gr/GCE film did not dissociate from the surface of GCE through the 10 CV cycles. The long term stability of the electrode was investigated by the CV measurement, after storage in the air, for one week at room temperature and the result showed that about 4% of its initial current was lost. The elevated stability of the film could be due to the Gr that was used as a substrate, which can improve the interaction between the GCE and highly conductive particles of Pd, so the film is strongly coated on the GCE. Another reason is that Gr shows good stability for the immobilization of Pd.
3.9.2. Interferences. Solutions containing more than one substance were considered to take into account different applications of the sensor. In this study, 5 μg of OMT and DCV was selected as a typical concentration. The maximum concentrations for the coexisting substances were determined when the substance caused a Pd/Gr/GCE intensity change of approximately ±3. We also studied the effect of inorganic ions like NH4+, Ca2+, Zn2+, Fe3+, SO42−, Cl−, Co32− and PO43−, and some other organophosphorous pesticides, such as parathion-methyl, trichlorfon and dichlorvos, and found that they did not interfere or affect the results. The interference of some metal ions, such as Pb2+, Hg2+, Cu2+ and Ag+, could be removed by an alkaline hydrolysis procedure.26 In our study, other organophosphorous pesticides under these optimized conditions were hardly detected, so the method for the determination of OMT and DCV is therefore very specific.
4. Conclusions
A new modified composite electrode was developed using a palladium (Pd) and graphene (Gr) nanocomposite. This can be used for the simultaneous determination of OMT and DCV in grain samples. The Pd/Gr/GCE nanocomposite was successfully characterised by SEM and CV, which indicated that the Pd nanoparticles were supported on the Gr/GCE surface. Furthermore, the synergistic effect of the Gr and Pd nanoparticles yielded lower LODs and improved the reproducibility, repeatability and the sensitivity of the composite electrode for the simultaneous determination of OMT and DCV. Additionally, the electrode showed long-term stability. The Pd/Gr/GCE composite electrode could an effective material for the simultaneous electrochemical determination of OMT and DCV and also as an alternative material to be used in environmental analysis.
Acknowledgements
This work was supported by the DAE-BRNS, Mumbai, India (no.: 2013/37C/22/BRNS) and they are gratefully acknowledged.
References
- S. Y. Wang, D. S. Yu, L. M. Dai, D. W. Chang and J.-B. Baek, ACS Nano, 2011, 5, 6202 CrossRef CAS PubMed.
- C. N. R. Rao, A. K. Sood, K. S. Subrahmanyam and A. Govindaraj, Angew. Chem., Int. Ed., 2009, 48, 7752 CrossRef CAS PubMed.
- K. S. Novoselov, A. K. Geim, S. V. Morozov, D. Jiang, M. I. Katsnelson, I. V. Grigorieva, S. V. Dubonos and A. A. Firsov, Nature, 2005, 438, 197 CrossRef CAS PubMed.
- B. Wang, J. Park, Ch. Y. Wang, H. Ahn and G. X. Wang, Electrochim. Acta, 2010, 55, 6812 CrossRef CAS PubMed.
- Z. Liu, B. Zhao, C. Guo, Y. Sun, Y. Shi, H. Yang and Z. Li, J. Colloid Interface Sci., 2010, 351, 233 CrossRef CAS PubMed.
- Y.-H. Qin, H. H. Yang, X.-S. Zhang, P. Li, X.-G. Zhou, L. Niu and W. K. Yuan, Carbon, 2010, 48, 3323 CrossRef CAS PubMed.
- Y. Wang, Z. Min, H. Yang, S. Ping and C. Ming, Int. J. Hydrogen Energy, 2010, 35, 10087 CrossRef CAS PubMed.
- S. Park, J. An, I. Jung, R. D. Piner, S. J. An, X. Li, A. Velamakanni and R. S. Ruoff, Nano Lett., 2009, 9, 1593–1597 CrossRef CAS PubMed.
- Y. Li, X. Fan, J. Qi, J. Ji, S. Wang, G. Zhang and F. Zhang, Nano Res., 2010, 3, 429–437 CrossRef CAS.
- J. Liu, W. Zhou, T. You, F. Li, E. Wang and S. Dong, Anal. Chem., 2010, 68, 3350–3353 CrossRef PubMed.
- Z. Liu, B. Zhao, C. Guo, Y. Sun, Y. Shi, H. Yang and Z. Li, J. Colloid Interface Sci., 2010, 351, 233–238 CrossRef CAS PubMed.
- A. F. Littke and G. C. Fu, Angew. Chem., Int. Ed., 2002, 41, 4176–4211 CrossRef CAS.
- B. Lim, M. Jiang, J. Tao, P. H. C. Camargo, Y. Zhu and Y. Xia, Adv. Funct. Mater., 2009, 19, 189–200 CrossRef CAS.
- M. T. Reetz and E. Westermann, Angew. Chem., Int. Ed., 2000, 9, 165–168 CrossRef.
- M. R. Kim and S. H. Choi, J. Nanomater., 2009 DOI:10.1155/2009/302919.
- Y. Nishihata, J. Mizuki, H. Tanaka, M. Uenishi and M. Kimura, J. Phys. Chem. Solids, 2005, 66, 274–282 CrossRef CAS PubMed.
- Y. Xiong, J. M. Mc Lellan, J. Chen, Y. Yin, Z. Y. Li and Y. Xia, J. Am. Chem. Soc., 2005, 127, 17118–17127 CrossRef CAS PubMed.
- L. Meng, J. Jin, G. Yang, T. Lu, H. Zhang and C. Cai, Anal. Chem., 2009, 81, 7271–7280 CrossRef CAS PubMed.
- M. Rajkumar, B. Devadas, S.-M. Chen and P.-C. Yeh, Colloids Surf., A, 2014, 452, 39–45 CrossRef CAS PubMed.
- Q. Wang, X. Cui, W. Guan, W. Zheng, J. Chen, X. Zheng, X. Zhang, C. Liu, T. Xue, H. Wang, Z. Jin and H. Teng, J. Phys. Chem. Solids, 2013, 74, 1470–1474 CrossRef CAS PubMed.
- S. Palanisamy, S. Ku and S.-M. Chen, Microchim. Acta, 2013, 180, 1037–1042 CrossRef CAS.
- H. Liu, X. Chen, L. Huang, J. Wang and H. Pan, Electroanalysis, 2014, 26, 556–564 CrossRef CAS.
- G. Chang, Y. Luo, W. Lu, X. Qin, A. M. Asiri, A. O. Al-Youbi and X. Sun, Am. J. Nanotechnol., 2013, 4(1), 1–7 CrossRef.
- J.-J. Shi and J.-J. Zhu, Electrochim. Acta, 2011, 56, 6008–6013 CrossRef CAS PubMed.
- Z. Zheng, Y. Du, Q. Feng, Z. Wang and C. Wang, J. Mol. Catal. A: Chem., 2012, 353–354, 80–86 CrossRef CAS PubMed.
- W. Yu, S. Yuansi, H. Junhua, Z. Wuming and Z. Xingyao, Wuhan Univ. J. Nat. Sci., 1998, 3(4), 469–472 CrossRef.
- J. A. G. Roach and L. J. Carson, J.-Assoc. Off. Anal. Chem., 1987, 70(3), 439–442 CAS.
- J. R. Ferreira, M. M. Faleao and A. Tainha, J. Agric. Food Chem., 1987, 35(4), 506–508 CrossRef CAS.
- W. Drescher and L. Fiedler, Mothode zum Nachweis yon Ruckstanden der, Chemosphere, 1983, 12, 1605–1610 CrossRef CAS.
- W. Yu, S. Yuansi, H. Junhua, Z. Wuming and Z. Xingyao, Wuhan Univ. J. Nat. Sci., 1998, 3(4), 469–472 CrossRef.
- J. Ngeh-Ngwainbi, A. A. Suleiman and G. G. Guilbault, Biosens. Bioelectron., 1990, 5, 13 CrossRef CAS.
- L. V. Rajakovic and S. Strbac, Anal. Chim. Acta, 1995, 315, 83 CrossRef CAS.
- Y. Tomita and G. G. Guilbault, Anal. Chem., 1980, 52, 1484 CrossRef CAS.
- G. G. Guilbault, J. Affolter and Y. Tomita, Anal. Chem., 2057, 1981, 53 Search PubMed.
- F. Cheng, W. Xianbao and Z. Wuming, Talanta, 1999, 49, 253–259 CrossRef CAS.
- D. De Souza and S. A. S. Machado, Anal. Bioanal. Chem., 2005, 382, 1720–1725 CrossRef CAS PubMed.
- Y. Bai, J. Chen, H. Mu, C. Zhang and B. Li, J. Agric. Food Chem., 2009, 57, 6238–6245 CrossRef CAS PubMed.
- Y. Tao, Y. Wang, L. Ye, H. Li and Q. Wang, Bull. Environ. Contam. Toxicol., 2008, 81, 210–215 CrossRef CAS PubMed.
- M. Sivaprasad, C. Swarupa, M. Dhananjayulu, M. R. Jayapal and N. Y. Sreedhar, J. Anal. Bioanal. Technol., 2014, 5, 192, DOI:10.4172/2155-9872.1000192.
- L. Han and X. Zhang, Electroanalysis, 2009, 21, 124 CrossRef CAS.
|
This journal is © The Royal Society of Chemistry 2015 |
Click here to see how this site uses Cookies. View our privacy policy here.