DOI:
10.1039/C4RA14456B
(Paper)
RSC Adv., 2015,
5, 21634-21639
Eu3+:Y2O3@CNTs—a rare earth filled carbon nanotube nanomaterial with low toxicity and good photoluminescence properties
Received
13th November 2014
, Accepted 10th February 2015
First published on 11th February 2015
Abstract
Red-emission phosphor europium-doped yttria (Eu3+:Y2O3) nanoparticles have been successfully filled into the nanocavity of carbon nanotubes (CNTs) via supercritical reaction and supercritical fluids followed by calcination. The existence of Eu3+:Y2O3 nanoparticles inside CNTs was characterized by TEM, EDS and XRD. The as-prepared nanomaterials (Eu3+:Y2O3@CNTs) exhibited strong red-emission at 610 nm, which corresponded to 5D0 → 7F2 transition within Eu3+ ions. It showed that the existence of the walls of the CNTs did not quench the luminescence of Eu3+:Y2O3. Due to the surface modification with Tween 80, Eu3+:Y2O3@CNTs had good water solubility. In vitro cytotoxicity studies showed that the as-prepared nanomaterials had low toxicity on HeLa cells at concentrations of 10–1000 μg mL−1. And their use as luminescence probes for live cell imaging was demonstrated by using inverted fluorescence microscopy. With the advantages of the easy dispersion in water, low toxicity, and good photoluminescence (PL) properties, the as-prepared Eu3+:Y2O3@CNTs could potentially be used as nanophosphors in bio-imaging.
Introduction
Benefited from their unique electronic, magnetic, and chemical properties arising from the 4f electrons,1 rare-earth compounds have drawn great research attention in recent years.2,3 Among the family of rare earth compounds, yttrium oxide (Y2O3) is a common used luminescent host material,4 and Eu3+:Y2O3 is considered to be one of the most promising red phosphors due to its excellent luminescence performance,5,6 which has practical applications in high quality fluorescence lamps,7 emissive displays,8 in vivo biological imaging9 and so on. For the application of luminescent probes, the water-solubility and biocompatibility of nanoparticles are important.
Unfortunately, despite recent advances in synthetic methods for controlling the size and shape of Eu3+:Y2O3 nanoparticles,5,10–20 many Eu3+:Y2O3 nanoparticles synthesized by a solution method are not directly used as biolabels due to their hydrophobic surface ligands, leading to their very low water-solubility. Additionally, the biological safety of the rare-earth nanomaterials remains controversial.21–24 For instance, T. Andelman et al. demonstrated that the Y2O3 nanoparticles could cause cytotoxicity by increasing the amount of reactive oxygen species (ROS).23 Sungho Lee et al. reported that the development of actin filament and cell proliferation could be inhibited by Y2O3 nanoparticles and the cytotoxicity of Y2O3 nanoparticles relied on their size.22 Therefore, it is necessary to develop a new and effective method to synthesize water-soluble or easily water-soluble and highly biocompatible Eu3+:Y2O3 nanoparticles for their application as biological fluorescent probes.
Carbon nanotubes (CNTs), which have hollow structures, can load functional materials inside the walls and combine the properties of CNTs with those of their guest components, to be used as delivery vehicles for drugs,25–28 bio-imaging29–31 and so on. The water-solubility of CNTs has been improved by sidewall covalent functionalization or noncovalent modification.32,33 Moreover, in recent years, researchers have performed a lot of work on the cytotoxicity of nanotubes34–49 and nanowires50–57 and found that water-soluble functionalized carbon nanotubes had low toxicity.48,49 Thus, CNTs can be used as an ideal carrier for rare-earth materials.
In this article, CNTs filled with Eu3+:Y2O3 nanoparticles were synthesized by a supercritical technique since this technique shows intriguing advantages for the synthesis of CNT-based composites, especially in filling materials into the nanocavity of CNTs.58,59 The property and structure of the as-synthesized nanomaterials were characterized by TEM, EDS, XRD, and fluorescence spectroscopy. In order to demonstrate the potential application of the synthesized nanomaterials in bio-imaging, we have further functionalized the nanomaterials with Tween 80, to render them water-soluble. In vitro cytotoxicity studies of the synthesized Eu3+:Y2O3@CNTs were also undertaken.
Materials and methods
Materials
Y(III) nitrate hexahydrate (99.9%) and Eu(III) nitrate hexahydrate (99.9%) were purchased from Aldrich Chemical Co. The CCK-8 Cell Counting Kit and MTT cell proliferation and cytotoxicity assay kit were purchased from Beyotime Biological Co. Ltd. The Dulbecco’s Modified Eagle’s Medium (DMEM) cell culture medium and Fetal bovine serum (FBS) were purchased from Gibico Biologicals Inc. All of the other chemicals were of analytical grade and purchased from Sinopharm Chemical Reagent Co. Ltd. and were used without further purification.
Material synthesis
Functionalization of the carbon nanotubes. The CNTs used in this work were purchased from Chengdu Organic Chemicals Co. Ltd. The outer diameter was 10–20 nm, and their average length was 0.5–2 μm. The CNTs were oxidized and cut by refluxing in 60% HNO3 for 12 h. After this reaction, the ends of the CNTs were opened and defects were formed on the side walls, resulting in carboxyl-containing CNTs.
Synthesis of Eu3+:Y2O3@CNT nanomaterials. Eu3+:Y2O3@CNTs were synthesized by a supercritical method, similar to the synthesis of Eu3+:Y2O3 nanoparticles,15 except that opened CNTs were added as the container for the rare-earth compounds. In a typical synthesis, 0.85 mmol of Y(NO3)3·6H2O and 0.15 mmol of Eu(NO3)3·6H2O were dissolved in 20 mL water and then mixed with 15 mL methanol. The mixed solution was put in a beaker under vigorous stirring for 10 min, and then the solution pH was adjusted to 7 by using 5 mL KOH solution. Finally, 40 mL of the above prepared mixture (volume ratio, water/methanol = 25/15) was transferred into a 100 mL stainless steel high-pressure reactor and mixed with 2 mg CNTs and heated at 400 °C for 10 min (the pressure was increased to 30 MPa). The filled samples were washed with distilled water for several times. The products were sonicated in 10 mL 65% HNO3 solution several times to remove the rare-earth compounds attached to the surface of the CNTs and washed with distilled water and ethanol for three times and dried in air at 60 °C for 6 h. The final products were obtained through a heat treatment at 1000 °C in Ar for 2 h. For comparison, Eu3+:Y2O3 nanoparticles were also synthesized by a similar method.
Noncovalent modification of Eu3+:Y2O3@CNTs with Tween 80. In order to obtain better water solubility, Tween 80 was used to modify the as-synthesized nanomaterials. Noncovalent modification of Eu3+:Y2O3@CNTs was performed as follows: the as-prepared Eu3+:Y2O3@CNT nanomaterials were sonicated in an aqueous solution with 1% (v/v) Tween 80 for 0.5 h, followed by centrifugation to remove large aggregates and bundled nanotubes. The free Tween 80 was then thoroughly removed by repeatedly filtrating the mixture through 8–14 kDa filters (Millipore). The Tween 80-functionalized Eu3+:Y2O3@CNTs were finally re-suspended in phosphate-buffered saline (PBS). The as-prepared Eu3+:Y2O3 nanoparticles and pristine CNTs were also modified with Tween 80 using the same method.
In vitro cytotoxicity studies of the as-prepared nanomaterials in water. The in vitro cytotoxicity of the as-synthesized nanomaterials was evaluated using two methods: the Cell Counting Kit-8 (CCK-8, Dojindo) and the MTT [3-(4,5-dimethylthiazol-2-yl)-2,5-diphenyltetrazolium bromide] assay. In the CCK-8 assay, HeLa cells were cultured in Dulbecco’s Modified Eagle’s Medium (DMEM) supplemented with 10% fetal bovine serum (FBS) and 1% penicillin–streptomycin. The cells were seeded in 96-well plates (1 × 104 cells per well). After 12 h of incubation, the medium was replaced with Tween 80-functionalized Eu3+:Y2O3@CNTs, Eu3+:Y2O3, and pristine CNTs, with different test concentrations (10, 100, 1000 μg mL−1) in the culture medium. After these materials were added, the incubations were carried out at 37 °C and in 5% CO2 atmosphere for another 12 h. Then the cell medium was removed and the dishes were washed by D-Hanks buffer solution for three times. One hundred microliters of CCK-8 solution were added to each well and incubated for an additional 2 h at 37 °C. The optical density (OD) of each well was recorded at 450 nm on a Microplate Reader (Infinite M200 Pro, A52769).In the MTT assay, 20 μL MTT was added to each well after the cells were exposed to the nanomaterials and incubated at 37 °C for 4 h. The formazan product was then dissolved in DMSO and the absorbance of each well was recorded at 570 nm wavelength.
The cell viability (% of control) is expressed as the percentage of (ODtest − ODblank)/(ODcontrol − ODblank), where ODtest is the optical density of the cells exposed to the nanomaterials, ODcontrol is the optical density of the cells and ODblank is the optical density of the wells without HeLa cells.
Cellular imaging. HeLa cells (1 × 104 cells) were seeded on glass coverslips that had been placed at the bottom of a culture dish. The HeLa cells were incubated for 12 h at 37 °C, and then the media was replaced by media that contained the Eu3+:Y2O3@CNTs (200 μL, 100 μg mL−1). After 6 h incubation, the cells were washed three times with an excess amount of PBS and directly imaged using an inverted fluorescence microscope (AMG EVOS f1).
Characterization. The morphology of the samples was observed using a transmission electron microscope (TEM, Tecnai G2 F20 U-TWIN) operating on 200 kV. The crystal structure of the sample was determined by X-ray diffraction analysis (XRD, Bruker D8 Advance) using graphite-monochromized Cu Kα radiation (λ = 1.5406 Å). Energy dispersive X-ray spectroscopy (EDS) spectra were collected on Tecnai G2 F20 with an accelerating voltage of 200 kV. The contents of Eu and Y were determined by inductively coupled plasma mass spectrometry (ICP-MS) (PerkinElmer, Nexlon 300D). The photoluminescence spectra were measured by a spectra fluorophotometer using a 450 W xenon lamp (Fluorolog-3 FL3-21) at room temperature. Cellular imaging was obtained using an inverted fluorescence microscope (AMG EVOS f1).
Results and discussion
Fig. 1 shows the TEM and high-resolution TEM (HRTEM) images of the as-synthesized Eu3+:Y2O3@CNTs (Fig. 1a and b) and Eu3+:Y2O3 (Fig. 1c and d). It can be seen from the TEM image that the Eu3+:Y2O3 nanoparticles have been successfully inserted into the nanocavity of the CNTs via the supercritical synthesis process. The size and aggregation of the Eu3+:Y2O3 nanoparticles filled in the CNTs were confined by the nanocavity of the CNTs compared to the free Eu3+:Y2O3 prepared under the same conditions (Fig. 1b and c). The HRTEM image (inset of Fig. 1b) shows that there were no Eu3+:Y2O3 nanoparticles on the outside walls of the CNTs. The restricted inner nanocavity of the CNTs prevents encapsulated Eu3+:Y2O3 nanoparticles from forming a large scale crystal (Fig. 1d).
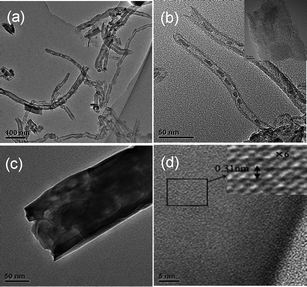 |
| Fig. 1 TEM and HRTEM images of the as-synthesized Eu3+:Y2O3@CNTs (a and b) and Eu3+:Y2O3 (c and d). | |
The Eu3+:Y2O3 encapsulated CNTs were also analysed by energy dispersive X-ray spectroscopy (Fig. 2). The presence of the Y and O elements attributed to Eu3+:Y2O3. Since the Eu3+:Y2O3 nanoparticles attached to the outer walls of the CNTs have been carefully removed with acid, the existence of Y elements further confirmed that the Eu3+:Y2O3 had been filled into the cavity of the CNTs. The peak of the Eu element was not appearing due to the low content of Eu in the as-prepared nanomaterials. The accurate content of the Eu and Y elements was detected by ICP-MS (Eu 9 wt%, Y 42 wt%), and the atom ratio of Eu and Y was found out to be 1
:
8.
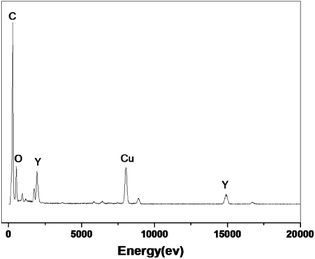 |
| Fig. 2 EDS analysis of the as-synthesized Eu3+:Y2O3@CNTs. | |
In order to further investigate the crystal structure of the as-synthesized nanomaterials, XRD was employed. Fig. 3 shows the XRD patterns of the pristine CNTs, Eu3+:Y2O3@CNTs and Eu3+:Y2O3. The XRD diffraction peaks confirmed the crystallinity of the as-synthesized nanomaterials. The peaks located at 2θ values 25.7 and 43.2 can be assigned to the (002) and (100) diffractions of the CNTs, whereas the strong peaks located at 2θ values 20.4, 29.0, 33.7, 48.4 and 57.6 are considered to be the (211), (222), (400), (440) and (622) diffractions of cubic Y2O3 (JCPDS File 70-0603), respectively. The XRD pattern of the as-synthesized Eu3+:Y2O3@CNT nanomaterials exhibits both diffractions of the CNTs and Y2O3, and confirms the successful filling of Eu3+:Y2O3 nanoparticles into the nanocavity of the CNTs.
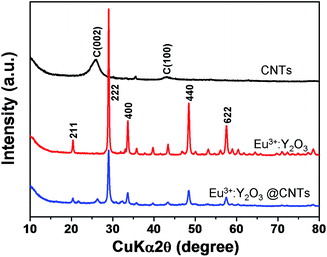 |
| Fig. 3 XRD patterns of pristine CNTs, Eu3+:Y2O3, and Eu3+:Y2O3@CNTs. | |
The room temperature photoluminescence (PL) emission spectra of the as-prepared Eu3+:Y2O3@CNT nanomaterials and pristine CNTs are shown in Fig. 4. The strongest peak of the Eu3+:Y2O3@CNTs at 610 nm excited at 254 nm of UV-light corresponds to the forced electric dipole transition (5D0 → 7F2) within the Eu3+ ions, which is the typical spectral property reported for Eu3+:Y2O3 nanoparticles.24 The peaks at 587, 592 and 599 nm are due to 5D0 → 7F1 transition, which are similar to those of Eu3+:Y2O3 nanoparticles synthesized using a supercritical method.15 The pristine CNTs show no peak between 570 nm and 670 nm under the same excitation conditions. Therefore, it can be concluded that the PL emission of the Eu3+:Y2O3 nanoparticles encapsulated in the hollow space of the CNTs is not quenched by the walls of the CNTs.
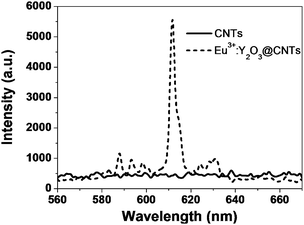 |
| Fig. 4 Photoluminescence spectra of Eu3+:Y2O3@CNTs and pristine CNTs excited at 254 nm. | |
For the potential applications as probes for bio-imaging, the cytotoxicity of the as-synthesized nanomaterials was investigated. The cytotoxicity of Tween 80-functionalized Eu3+:Y2O3@CNTs was investigated in HeLa cells using CCK-8 and MTT assays. The viability of the untreated cells was chosen as the control. Fig. 5 shows the viability of HeLa cells treated by functionalized Eu3+:Y2O3@CNTs, Eu3+:Y2O3 and pristine CNTs at concentrations in the range of 10–1000 μg mL−1. The results show a dose dependent decrease in their relative cell viability with the concentrations of the composite increased. The Eu3+:Y2O3@CNTs show low toxicity to HeLa cells even when the cells were exposed to a high concentration of 1000 μg mL−1 for a period of 12 h while Eu3+:Y2O3 shows a rather higher cytotoxicity in the same concentration, which indicate that after encapsulated by CNTs the cytotoxicity of Eu3+:Y2O3 nanoparticles is reduced. The above results suggest that the as-synthesized Eu3+:Y2O3@CNTs can be used as potential probes for bio-imaging. The results also indicated that pristine CNTs are more toxic to HeLa cells than Eu3+:Y2O3 filled CNTs in the same weight concentration. According to the results of ICP-MS, the mass ratio of Eu and Y in Eu3+:Y2O3@CNTs was around 50%, and the number of CNTs in pristine CNTs was much more than in Eu3+:Y2O3@CNTs at the same weight.
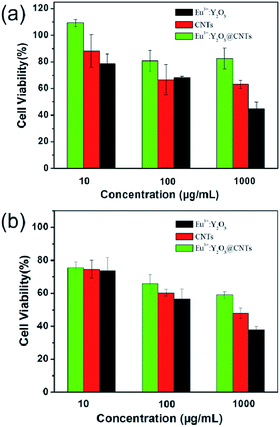 |
| Fig. 5 Cellular viabilities of HeLa cells exposed to functionalized Eu3+:Y2O3@CNTs, CNTs, and Eu3+:Y2O3 nanoparticles with different concentrations determined by CCK-8 assay (a) and MTT assay (b). | |
To demonstrate the potential of the as-prepared Eu3+:Y2O3@CNTs as probes for biological imaging, we incubated amphiphilic Tween 80-functionalized Eu3+:Y2O3@CNTs with HeLa cells. The fluorescence image (Fig. 6) shows that the Eu3+:Y2O3@CNTs exhibit good photoluminescence properties inside the cells.
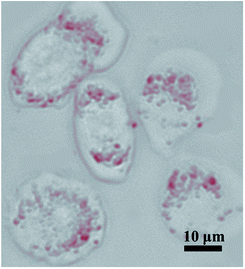 |
| Fig. 6 Overlay image of live HeLa cells after incubation with Eu3+:Y2O3@CNT nanomaterials (100 μg mL−1) (red represents the emission from the Eu3+:Y2O3@CNTs). | |
Conclusions
In summary, Eu3+:Y2O3 filled CNT nanomaterials have been successfully synthesized by a supercritical technique, which is the first case that supercritical synthesis is used in the nanocavity of nanotubes. The as-synthesized Eu3+:Y2O3@CNTs were characterized by TEM, EDS, XRD and PL spectroscopy. With the excitation at 254 nm of UV-light, a special red-emission at 610 nm has been observed, which was from the Eu3+:Y2O3 nanoparticles encapsulated in the CNTs. Surface functionalization with Tween 80 enabled the synthesized materials to be dissolved in water and to have the possibility of further functionalization by other biomolecules. The Eu3+:Y2O3@CNT nanomaterials show low cytotoxicity in HeLa cells at concentrations of 10–1000 μg mL−1. Using an inverted fluorescence microscope, we have demonstrated the application of Eu3+:Y2O3@CNTs as luminescent probes for live cells. With the properties of fluorescence emission, easily water-soluble and low cytotoxicity, the Eu3+:Y2O3@CNT nanomaterials have potential applications in bio-imaging. The supercritical synthesis method in filling the inner space of the CNTs could be applied to other rare-earth materials, such as up-conversion materials, which have greater potential in deep-tissue imaging.
Acknowledgements
The research was supported by National Basic Research Program of China (973 Program) (2012CB932601) and National Natural Science Foundation of China (21271174).
Notes and references
- A. W. Xu, Y. Gao and H. Q. Liu, J. Catal., 2002, 207, 151–157 CrossRef CAS.
- Q. Liu, W. Feng and F. Li, Coord. Chem. Rev., 2014, 273, 100–110 CrossRef PubMed.
- S. Gai, C. Li, P. Yang and J. Lin, Chem. Rev., 2014, 114, 2343–2389 CrossRef CAS PubMed.
- A. O. G. Dikovska, P. A. Atanasov, M. J. de Castro, A. Perea, J. Gonzalo, C. N. Afonso and J. G. Lopez, Thin Solid Films, 2006, 500, 336–340 CrossRef CAS PubMed.
- Z. Fu, S. Zhou, T. Pan and S. Zhang, J. Lumin., 2007, 124, 213–216 CrossRef CAS PubMed.
- S. Dutta, Q. Mohammad and S. S. Manoharan, J. Mater. Sci. Lett., 2002, 21, 1077–1079 CrossRef CAS.
- C. R. Ronda, J. Alloys Compd., 1995, 225, 534–538 CrossRef CAS.
- C. H. Kim, I. E. Kwon, C. H. Park, Y. J. Hwang, H. S. Bae, B. Y. Yu and C. H. Pyun, J. Alloys Compd., 2000, 311, 33–39 CrossRef CAS.
- J. Feng, G. M. Shan, A. Maquieira, M. E. Koivunen, B. Guo, B. D. Hammock and I. M. Kennedy, Anal. Chem., 2003, 75, 5282–5286 CrossRef CAS.
- S. M. Rafiaei, A. Kim and M. Shokouhimehr, Nanosci. Nanotechnol. Lett., 2014, 6, 692–696 CrossRef CAS PubMed.
- T. Chen and Y. Xia, Mater. Sci. Semicond. Process., 2014, 19, 1–5 CrossRef CAS PubMed.
- P. Psuja, W. Strek and I. Yelkin, J. Nanopart. Res., 2014, 16, 2176 CrossRef PubMed.
- H. Huang, H. Zhang, W. Zhang, S. Lian, Z. Kang and Y. Liu, J. Lumin., 2012, 132, 2155–2160 CrossRef CAS PubMed.
- X. Zhang, J. Wang, K. Guo, H. Chen, X. Yang and J. Zhao, J. Alloys Compd., 2012, 517, 149–156 CrossRef CAS PubMed.
- M. K. Devaraju, S. Yin and T. Sato, Inorg. Chem., 2011, 50, 4698–4704 CrossRef CAS PubMed.
- M. K. Devaraju, S. Yin and T. Sato, J. Nanosci. Nanotechnol., 2010, 10, 731–738 CrossRef CAS PubMed.
- M. K. Devaraju, S. Yin and T. Sato, Mater. Sci. Eng., C, 2009, 29, 1849–1854 CrossRef CAS PubMed.
- J. Dhanaraj, R. Jagannathan, T. R. N. Kutty and C. H. Lu, J. Phys. Chem. B, 2001, 105, 11098–11105 CrossRef CAS.
- A. Konrad, T. Fries, A. Gahn, F. Kummer, U. Herr, R. Tidecks and K. Samwer, J. Appl. Phys., 1999, 86, 3129–3133 CrossRef CAS PubMed.
- D. K. Williams, B. Bihari, B. M. Tissue and J. M. McHale, J. Phys. Chem. B, 1998, 102, 916–920 CrossRef CAS.
- Z.-L. Wang, J. Hao, H. L. W. Chan, G.-L. Law, W.-T. Wong, K.-L. Wong, M. B. Murphy, T. Su, Z. H. Zhang and S. Q. Zeng, Nanoscale, 2011, 3, 2175–2181 RSC.
- S. Lee, T. Kasuga and K. Kato, J. Ceram. Soc. Jpn., 2010, 118, 428–433 CrossRef CAS.
- T. Andelman, S. Gordonov, G. Busto, P. V. Moghe and R. E. Riman, Nanoscale Res. Lett., 2010, 5, 263–273 CrossRef CAS PubMed.
- G. K. Das and T. T. Y. Tan, J. Phys. Chem. C, 2008, 112, 11211–11217 CAS.
- L. Jian, Y. Siew Qi, Y. Sia Lee, T. R. Nayak, G. W. Chandra, A. Wee Han, T. Panczyk, S. Ramaprabhu, S. K. Vashist, S. Fwu-Shan, A. Tan and G. Pastorin, Carbon, 2012, 50, 1625–1634 CrossRef PubMed.
- Z. Su, S. Zhu, A. D. Donkor, C. Tzoganakis and J. F. Honek, J. Am. Chem. Soc., 2011, 133, 6874–6877 CrossRef CAS PubMed.
- Y. Ren and G. Pastorin, Adv. Mater., 2008, 20, 2031–2036 CrossRef CAS.
- S. Hampel, D. Kunze, D. Haase, K. Kraemer, M. Rauschenbach, M. Ritscbel, A. Leonbardt, J. Thomas, S. Oswald, V. Hoffmann and B. Buechner, Nanomedicine, 2008, 3, 175–182 CrossRef CAS PubMed.
- L. Maggini, J. Mohanraj, H. Traboulsi, A. Parisini, G. Accorsi, N. Armaroli and D. Bonifazi, Chem.–Eur. J., 2011, 17, 8533–8537 CrossRef CAS PubMed.
- S. Y. Hong, G. Tobias, K. T. Al-Jamal, B. Ballesteros, H. Ali-Boucetta, S. Lozano-Perez, P. D. Nellist, R. B. Sim, C. Finucane, S. J. Mather, M. L. H. Green, K. Kostarelos and B. G. Davis, Nat. Mater., 2010, 9, 485–490 CrossRef CAS PubMed.
- B. Sitharaman, K. R. Kissell, K. B. Hartman, L. A. Tran, A. Baikalov, I. Rusakova, Y. Sun, H. A. Khant, S. J. Ludtke, W. Chiu, S. Laus, E. Toth, L. Helm, A. E. Merbach and L. J. Wilson, Chem. Commun., 2005, 3915–3917 RSC.
- H. Sun, P. She, G. Lu, K. Xu, W. Zhang and Z. Liu, J. Mater. Sci., 2014, 49, 6845–6854 CrossRef CAS.
- D. Tasis, N. Tagmatarchis, A. Bianco and M. Prato, Chem. Rev., 2006, 106, 1105–1136 CrossRef CAS PubMed.
- S. Arora, H. Kaur, R. Kumar, R. Kaur, D. Rana, C. S. Rayat, I. Kaur, S. K. Arora, P. Bubber and L. M. Bharadwaj, Fullerenes, Nanotubes, Carbon Nanostruct., 2015, 23, 377–382 CrossRef CAS.
- M. Song, F. Wang, L. Zeng, J. Yin, H. Wang and G. Jiang, Environ. Sci. Technol., 2014, 48, 13978–13984 CrossRef CAS PubMed.
- L. M. Saeed, M. Mahmood, S. J. Pyrek, T. Fahmi, Y. Xu, T. Mustafa, Z. A. Nima, S. M. Bratton, D. Casciano, E. Dervishi, A. Radominska-Pandya and A. S. Biris, J. Appl. Toxicol., 2014, 34, 1188–1199 CrossRef CAS PubMed.
- B. M. Rotoli, P. Guidi, B. Bonelli, M. Bernardeschi, M. G. Bianchi, S. Esposito, G. Frenzilli, P. Lucchesi, M. Nigro, V. Scarcelli, M. Tomatis, P. P. Zanello, B. Fubini, O. Bussolati and E. Bergamaschi, Chem. Res. Toxicol., 2014, 27, 1142–1154 CrossRef CAS PubMed.
- J. S. M. Nithya and A. Pandurangan, RSC Adv., 2014, 4, 32031–32046 RSC.
- S. Alarifi, D. Ali, A. Verma, F. N. Almajhdi and A. A. Al-Qahtani, In Vitro Cell. Dev. Biol.: Anim., 2014, 50, 714–722 CrossRef CAS PubMed.
- Y. Mohammadian, S. J. Shahtaheri, A. A. S. Yaraghi, H. Kakooei and M. Hajaghazadeh, Toxicol. Environ. Chem., 2013, 95, 1037–1047 CrossRef CAS.
- C. Meindl, M. Absenger, E. Roblegg and E. Frohlich, BioMed Res. Int., 2013, 2013, 564804 Search PubMed.
- C. Li, S. Bolisetty, K. Chaitanya, J. Adamcik and R. Mezzenga, Adv. Mater., 2013, 25, 1010–1015 CrossRef CAS PubMed.
- E. Froehlich, C. Meindl, A. Hoefler, G. Leitinger and E. Roblegg, Nanotoxicology, 2013, 7, 1211–1224 CrossRef CAS PubMed.
- T. Dong, Y. Ji and J. Wang, Asian J. Ecotoxicol., 2013, 8, 55–60 CAS.
- J. Yuan, H. Gao, J. Sui, H. Duan, W. N. Chen and C. B. Ching, Toxicol. Sci., 2012, 126, 149–161 CrossRef CAS PubMed.
- D. Cavallo, C. Fanizza, C. L. Ursini, S. Casciardi, E. Paba, A. Ciervo, A. M. Fresegna, R. Maiello, A. M. Marcelloni, G. Buresti, F. Tombolini, S. Bellucci and S. Iavicoli, J. Appl. Toxicol., 2012, 32, 454–464 CrossRef CAS PubMed.
- J. Yuan, H. Gao, J. Sui, W. N. Chen and C. B. Ching, Toxicol. in Vitro, 2011, 25, 1820–1827 CrossRef CAS PubMed.
- Y. Liu, Y. Zhao, B. Sun and C. Chen, Acc. Chem. Res., 2013, 46, 702–713 CrossRef CAS PubMed.
- K. Kostarelos, A. Bianco and M. Prato, Nat. Nanotechnol., 2009, 4, 627–633 CrossRef CAS PubMed.
- W.-S. Lin, H.-M. Lin, H.-H. Chen, Y.-K. Hwu and Y.-J. Chiou, J. Nanomater., 2013, 237439 Search PubMed.
- N. K. Verma, J. Conroy, P. E. Lyons, J. Coleman, M. P. O’Sullivan, H. Kornfeld, D. Kelleher and Y. Volkov, Toxicol. Appl. Pharmacol., 2012, 264, 451–461 CrossRef CAS PubMed.
- Z. Ji, X. Wang, H. Zhang, S. Lin, H. Meng, B. Sun, S. George, T. Xia, A. E. Nel and J. I. Zink, ACS Nano, 2012, 6, 5366–5380 CrossRef CAS PubMed.
- M. Safi, M. Yan, M.-A. Guedeau-Boudeville, H. Conjeaud, V. Garnier-Thibaud, N. Boggetto, A. Baeza-Squiban, F. Niedergang, D. Averbeck and J.-F. Berret, ACS Nano, 2011, 5, 5354–5364 CrossRef CAS PubMed.
- G. Ning, W. Hongjun and Y. Eui-Hyeok, Nanotechnology, 2010, 21, 105107 CrossRef PubMed.
- S. Meng-Meng, S. Wen-Jing, B. Hong, W. Jun, W. Wei-Lin, S. Jun and Y. Min, Biomaterials, 2010, 31, 1509–1517 CrossRef PubMed.
- M. Lv, S. Su, Y. He, Q. Huang, W. Hu, D. Li, C. Fan and S.-T. Lee, Adv. Mater., 2010, 22, 5463–5467 CrossRef CAS PubMed.
- D. C. Julien, C. C. Richardson, M. F. Beaux, II, D. N. McIlroy and R. A. Hill, J. Nanomed. Nanotechnol., 2010, 6, 84–92 CrossRef CAS PubMed.
- J. Hao, L. Guan, X. Guo, Y. Lian, S. Zhao, J. Dong, S. Yang, H. Zhang and B. Sun, J. Nanosci. Nanotechnol., 2011, 11, 7857–7862 CrossRef CAS PubMed.
- H. Jian, L. Yongfu, G. Lunhui, Y. Dongmei, G. Xihong, Z. Shixiong, Z. Yuliang, K. Ibrahim, W. Jiaou, Q. Haijie, D. Jinquan, Y. Hui, X. Gengmei and S. Baoyun, Nanoscale, 2011, 3, 3103–3108 RSC.
|
This journal is © The Royal Society of Chemistry 2015 |
Click here to see how this site uses Cookies. View our privacy policy here.