DOI:
10.1039/C5GC00402K
(Paper)
Green Chem., 2015,
17, 3000-3009
Fluorine gas for life science syntheses: green metrics to assess selective direct fluorination for the synthesis of 2-fluoromalonate esters†
Received
19th February 2015
, Accepted 17th March 2015
First published on 17th March 2015
Abstract
Optimisation and real time reaction monitoring of the synthesis of 2-fluoromalonate esters by direct fluorination using fluorine gas is reported. An assessment of green metrics including atom economy and process mass intensity factors, demonstrates that the one-step selective direct fluorination process compares very favourably with established multistep processes for the synthesis of fluoromalonates.
Introduction
Green metrics demonstrate for the first time that direct fluorination processes using fluorine gas can compete favourably from an environmental point of view, with established halogen exchange methodology for the synthesis of important fluorinated building blocks. Life-science products containing fluorine atoms are of increasing importance and many commercially significant pharmaceuticals and agrochemicals owe their activity, in part, to the presence of fluorinated structural subunits.1 The FDA lists over 150 active pharmaceutical ingredients which contain fluorinated motifs that are used in many therapeutic areas2 because fluorine incorporation into organic systems can lead to a variety of physical, chemical and biological activity changes depending upon the system such as increasing metabolic stability, increasing lipophilicity and changing pKa of adjacent receptor sites.1
Whilst many fluorinated life science products contain fluoro- or trifluoromethyl-aromatic units, drug development programmes now require chemical entities where fluorine atoms are located at less accessible sites and, consequently, there continues to be great interest for the development of efficient, selective and economically viable fluorination methodologies for industry.3 The commitment by many pharmaceutical companies to significantly reduce waste streams and the associated carbon footprint during drug manufacture provides a further requirement for the development of more environmentally benign, process intensive and reliable fluorination methods for large scale synthesis.
Recently, the concept of green chemistry has found wide-spread recognition in the chemical industries and various green chemistry related programs have been initiated both within and between major industrial companies. For example, several pharmaceutical companies now provide publicly available information on their solvent and reagent selection guides that were largely developed by their respective green chemistry groups.4 To assess the green credentials of a reaction, various mass balance based metrics have been developed and E factor, reaction mass efficiency (RME) and process mass intensity (PMI) are routinely assessed for process development campaigns. Such guides and metrics along with environmental, health and safety assessments form the basis of green metrics packages for the comparative assessment of alternative synthetic methods.5
Large scale manufacture of most fluorinated systems are carried out using inexpensive anhydrous hydrogen fluoride (aHF) by, for example, very well established multi-step Balz-Schiemann6 and halogen exchange (Halex)7 processes respectively and subsequent functional group transformation of the resulting fluorinated aromatic building blocks. Whilst the use of aHF in industry is widespread, the highly corrosive, acidic nature of this reagent precludes fluorination reactions to appropriate, structurally simple organic substrates that are pre-functionalised by nitration or chlorination by, in many cases, multi-step procedures.
In principle, the most direct, least wasteful and economically viable method of fluorine introduction for large scale synthesis is the selective conversion of carbon–hydrogen bonds to carbon–fluorine bonds using inexpensive fluorine gas.8 However, despite advances in selective direct fluorination methodology, involving both batch and flow protocols, the use of fluorine gas for life science product manufacturing has so far been limited to the synthesis of 5-fluorouracil9 and a key intermediate for the synthesis of Voriconazole (V-Fend, Pfizer).10 However, although electrochemical cells used for the production of fluorine gas11 are in operation in many silicon-wafer fabrication plants,12 precluding the use of fluorine gas in high pressure cylinders, selective direct fluorination technology has not been widely adopted by the life science industries.
The Durham group has reported the selective fluorination of various 1,3-diketones and ketoesters by both batch13 and continuous flow processes.14 Additionally, the synthesis of diethyl 2-fluoromalonate by direct fluorination has been previously achieved in good conversion, but the selectivity of the reaction was reported to be relatively low.15 Dialkyl 2-fluoromalonate esters could, in principle, be very useful fluorinated building blocks for the synthesis of fluorinated derivatives, and various alkylation,16 Michael addition17 and heterocycle formation18 processes have been described, providing an indication of the potential synthetic utility of this multi-functional, selectively fluorinated system.19 A growing number of patents utilising fluoromalonate as a substrate for the synthesis of a range of biologically active systems have been published recently20 and reviewed.19 For example, Fluoxastrobin (Fandango®), a fungicide marketed by Bayer CropScience that has achieved global annual sales of over €140 m since its launch in 2005,21 and TAK-733, an anti-cancer drug candidate,22 employ 2-fluoromalonate esters as the key fluorinated starting material (Scheme 1).
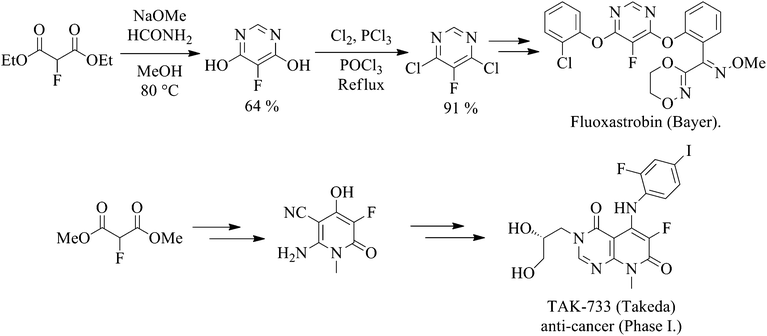 |
| Scheme 1 2-Fluoromalonate esters used in the synthesis of Fluoxastrobin and TAK-733. | |
There are three realistic, low-cost synthetic strategies available for the large scale manufacture of diethyl 2-fluoromalonate ester (Scheme 2) which involve reaction of ethanol with hexafluoropropene (HFP),23 halogen exchange (Halex)24 and selective direct fluorination15 processes. Other syntheses of fluoromalonate esters using electrophilic fluorinating agents such as Selectfluor™ are possible, but are not sufficiently commercially attractive to be considered for manufacture on the large scale.
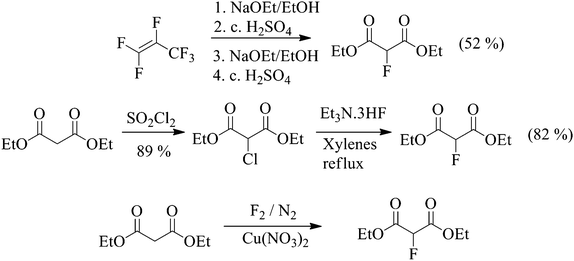 |
| Scheme 2 Synthetic routes to 2-fluoromalonate esters. | |
In this paper, we reassess and optimise the direct fluorination of diethyl malonate, catalysed by copper nitrate, with a view to intensifying this transformation and reducing its overall environmental impact. Upon optimisation, a comparison of the green metrics of selective direct fluorination with corresponding literature HFP and Halex routes for the synthesis of fluoromalonates to determine the relative merits of the three possible routes.
Results and discussion
Before a comparison of the green metrics between the three possible, economically viable large scale processes for the synthesis of fluoromalonate esters (Scheme 2) could be carried out, some primary goals for the optimisation of the process were targeted: complete conversion of the starting material is essential because it can be difficult to separate the starting material from the desired monofluorinated product by simple distillation; fluorine gas usage should be minimised because neutralisation of excess reagent could potentially generate significant amounts of waste; reduction in volumes of solvents used to reduce waste streams and overall intensification of the fluorination process and replacement and/or reduction of all environmentally harmful solvents used.
Conventional batch direct fluorination reactions of malonate esters were carried out in glassware vessels by introduction of fluorine gas, as a 10% or 20% mixture in nitrogen (v/v), at a prescribed rate via a gas mass flow controller into a solution of malonate ester and copper nitrate catalyst in acetonitrile using equipment described previously.15c
To better understand the relationship between fluorine gas introduction and rate of conversion, real time IR spectroscopic monitoring of the reaction was chosen as the most suitable technique. The use of the ReactIR technique25 was enabled by a sufficient difference in the carbonyl group stretching frequencies (1734 cm−1 for diethyl malonate and 1775 cm−1 for diethyl 2-fluoromalonate) and provided an in situ reaction profile (Fig. 1).
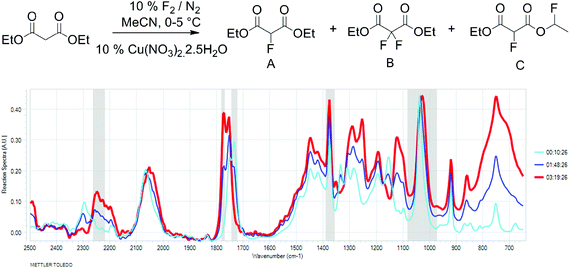 |
| Fig. 1 IR spectra of the fluorination reaction at 0% (light blue), 50% (dark blue) and 100% (red) conversions. | |
The real time reaction monitoring (Fig. 1 and 2) revealed that the reaction begins instantly upon initiation of fluorine introduction and the reaction conversion is directly proportional to the amount of fluorine gas passed into the reaction vessel. When the intensity of the fluoromalonate carbonyl peak (1775 cm−1) reached a maximum, the introduction of fluorine gas was stopped and the crude reaction mixture was analysed by 1H and 19F NMR spectroscopy. Complete conversion of the starting material was observed and diethyl fluoromalonate was formed with 93% selectivity after introducing 1.1 equivalents of fluorine into the reaction mixture. The small excess of fluorine explains the unexpectedly small amount of difluorinated side products B and C (4.5 and 2.5% respectively) which were the major impurities (6.5 and 9% respectively) when larger excess of fluorine gas (1.8 eq.) was used.
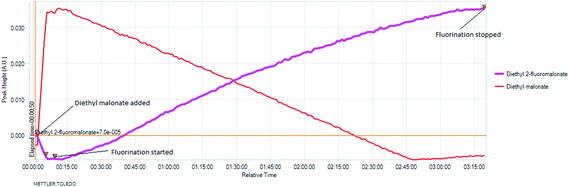 |
| Fig. 2
In situ monitoring of the fluorination of diethyl malonate. | |
The effect of concentration of fluorine in nitrogen, reaction temperature, copper nitrate catalyst loading and concentration of malonate substrate in acetonitrile were varied to optimise the fluorination process (Table 1). Additionally, reactions described in Table 1 allowed an assessment of various factors that have a major influence on the environmental impact of the process such as solvent usage, reaction temperature and the amount and composition of waste generated. In each case 20 mmol (3.20 g) of diethyl malonate was used as substrate and the isolated mass balance of crude material obtained after work-up was recorded along with the conversion of starting material and yield of fluorinated products (Table 1).
Table 1 Fluorination of diethyl malonate ester using fluorine gas catalysed by Cu(NO3)2·2.5H2O

|
Entry no. |
T/°C |
C
malonate (mol L−1) |
Catalyst (mol%) |
F
2 in N2 (% v/v) |
Conversion (1H NMR) |
A/B/C ratio (19F NMR) |
Isolated weight |
1 |
0–5 |
1.0 |
10 |
10 |
100% |
93.5/4.5/2 |
3.37 g |
2 |
0–5 |
1.5 |
10 |
10 |
100% |
94/4/2 |
3.30 g |
3 |
0–5 |
1.0 |
5 |
10 |
97% |
95/4/1 |
3.53 g |
4 |
0–5 |
1.0 |
2.5 |
10 |
82% |
95/4/1 |
3.51 g |
5 |
RT |
1.0 |
10 |
10 |
56% |
97.5/1.5/1 |
3.33 g |
6 |
0–5 |
1.0 |
10 |
15 |
85% |
97.5/1.5/1 |
3.47 g |
7 |
0–5 |
1.0 |
10 |
20 |
100% |
94/3/3 |
3.50 g |
8 |
0–5 |
2.0 |
5 |
20 |
52% |
92/5/3 |
3.40 g |
In all cases, small quantities of side products were formed which were identified by 19F NMR and these originate from two different processes: 3,3-difluoromalonate is produced from enolisation of diethyl fluoromalonate which is much slower than enolisation of the diethyl malonate substrate, while the fluoroethyl fluoromalonate is postulated to form via an electrophilic process.26
The data in Table 1 suggest that the concentration of the malonate ester substrate in acetonitrile has no apparent effect on the outcome of the reaction although solvent is required for these reactions because diethyl malonate does not dissolve the catalyst. Additionally, the use of high dielectric constant media, such as acetonitrile, have been found to be beneficial for the control of selectivity of electrophilic direct fluorination processes.27 For convenience, a 1.5 M concentration of malonate in acetonitrile was chosen as the optimal conditions which is approximately 5 mL solvent per 1 mL of diethyl malonate.
The concentration of fluorine gas, between 10–20% v/v in nitrogen, does not affect the selectivity of the reaction and the quality of the product either, as exemplified by the product mixtures obtained from reactions 1, 2 and 7 which have identical compositions. In contrast, carrying out fluorination reactions at room temperature rather than cooling the reaction mixture to 0–5 °C leads to increased catalyst decomposition which results in an insoluble copper species that on occasion blocked the fluorine gas inlet tube. In addition, without cooling, the exothermic nature of this fluorination reaction led to a slight reaction temperature increase (from 20 to 29 °C in a small scale laboratory experiment) resulting in loss of some solvent and some decomposition of the catalyst and product degradation.
Lowering the concentration of the copper nitrate catalyst led to a significantly slower reaction as would be expected and required the use of a larger excess of fluorine gas to enable sufficiently high conversion. For example, the reaction proceeded in the presence of only 2.5 mol% catalyst, but in this case 40% excess fluorine was required to reach 100% conversion.
Typical literature work-up procedures for direct fluorination reactions15 involve pouring the reaction mixture into 3 to 5 volumes of water and extracting the resulting mixture three times with dichloromethane. The combined organic fraction is typically washed with water, saturated sodium bicarbonate solution and dried over sodium sulfate before evaporation of the solvent to give the crude reaction product. We sought to improve the work-up to enable recycling of the reaction solvent and substitute the use of environmentally harmful dichloromethane in the reaction work-up stage. Upon completion of fluorine gas addition, acetonitrile was evaporated for reuse and then the residue was partitioned between ethyl acetate and water, the organic phase was washed with water, saturated Na2CO3 solution and saturated brine and dried prior to evaporation under reduced pressure. Modification of the workup procedure in this manner enables the recovery of acetonitrile and ethyl acetate and significantly reduces the amount of aqueous waste generated. When direct reuse of the recovered acetonitrile was attempted, a copper containing precipitate was formed presumably because of the high HF content of the solvent (0.63 M by titration). Therefore, before reuse of the solvent, HF must be removed. Stirring the recovered reaction solvent with solid Na2CO3 lowered the acid content to an acceptable level (0.04 M) and when a second fluorination reaction was carried out in the recovered, neutralised acetonitrile, no change in the fluorination reaction profile was observed.
Upon completion of these optimisation studies, selective fluorination reactions of malonate esters were scaled up to 40 g scale in the laboratory without experiencing any change in product profile. Isolation of significant quantities of monofluoromalonate A crude product (99% yield, 95% purity) was achieved which could be used in the subsequent cyclisation processes described below without further purification or, if high purity material was required, could be purified by fractional vacuum distillation (bp. 102–103 °C, 18 mbar) to produce 99% pure material in 77% yield.
Related malonate esters were also subjected to direct fluorination using the optimised conditions established above. In the case of di-tert-butyl malonate, fluorination was carried out on 12 g scale. 100% conversion was reached after the introduction of 1.2 equivalents of fluorine gas and the desired product was isolated in 96% yield. The purity of the crude product was higher than 97% by 1H and 19F NMR spectroscopy without any further purification and as expected, the only side product was the 2,2-difluorinated product (Scheme 3).
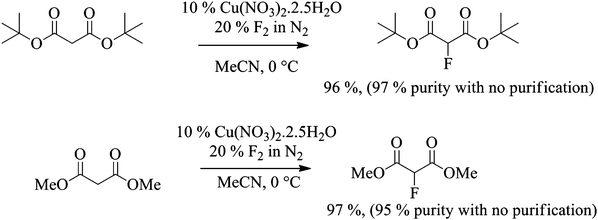 |
| Scheme 3 Fluorination of di-methyl and di-tert-butyl malonates. | |
From an atom economy point of view, methyl ester derivatives are preferable substrates compared to ethyl or other higher alkyl esters since they lead to smaller quantities of waste and so the fluorination of dimethyl malonate was investigated. Using 10% catalyst and 1.1 equivalent of fluorine, 20 g of dimethyl malonate was fluorinated to afford dimethyl fluoromalonate in 97% yield and 95% purity after isolation by simple work-up and no further purification. In this case, the only side product was dimethyl 2,2-difluoromalonate which was separated from dimethyl fluoromalonate by fractional distillation to afford high purity dimethyl fluoromalonate which crystallises at room temperature (Fig. 3).
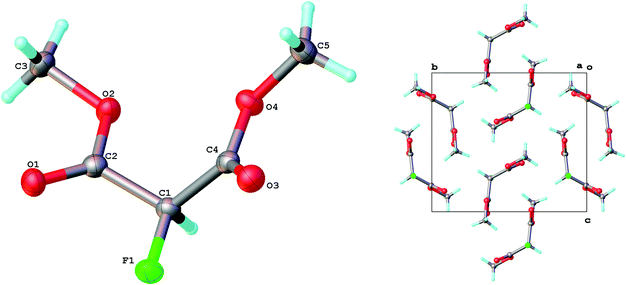 |
| Fig. 3 Dimethyl fluoromalonate crystal structure and packing. | |
Condensation of fluoromalonate esters with dinucleophiles is a convenient route to multifunctional fluorinated heterocyclic scaffolds. A number of nitrogen dinucleophiles were reacted with the crude diethyl fluoromalonate product synthesised above (∼95% purity) to afford pyrimidine and diazepane derivatives which could be purified readily by recrystallization and demonstrate the usefulness of 95% purity fluoromalonate material for further synthesis. Yields are unoptimised, but comparable to similar literature procedures.
In particular, crude diethyl fluoromalonate prepared above reacts efficiently with formamidine to give 5-fluoro-4,6-dihydroxypyrimidine, a key intermediate in the synthesis of Fluoxastrobin, in good yield (64%), which is comparable with previously reported 61–78% yields (Table 2).21a
Table 2 Synthesis of fluorinated heterocycles using crude (95% pure) diethyl fluoromalonate ester
To compare the metrics of the optimised direct fluorination process with other methods for the synthesis of fluoromalonate esters, a “first pass” metrics package, was implemented.28 Hexafluoropropene (HFP) is an important, inexpensive perfluorinated building block used for the synthesis of various well known fluoropolymers and refrigerant gases and is, therefore, available on the industrial scale.29 Synthesis of fluoromalonates by reaction of HFP with ethanol and H2SO4 affords good purity product in fair yield, but, from an environmental impact point of view, this is a very wasteful and low-atom economy process. Firstly, the synthesis of the starting material requires many steps, ultimately by reaction of low molecular weight hydrocarbons with chlorine gas and subsequent halogen exchange in a very energy intensive process3b and, secondly, a significant amount of hazardous, strongly acidic waste is generated in the reaction of HFP with ethanol.
The halogen exchange reaction requires the industrial synthesis of high purity chloromalonate ester since the purity of the final fluoromalonate product largely depends on the efficiency and selectivity of this step. The chlorination reaction with sulfuryl chloride is reasonably selective and pure diethyl chloromalonate can be obtained in good yield30 after vacuum distillation and it is reasonable to assume that this reaction is suitable for large scale synthesis. The halogen exchange reaction is also very efficient since HF.amine systems are convenient, reactive sources of fluoride ion and additionally, they can be handled safely since they are less volatile than aHF and are commercially available on the multi-tonne scale (Table 3).
Table 3 Green metrics to compare methods for the synthesis of diethyl fluoromalonate
Fluoromalonate synthesis |
HFP method |
Halex cumulative |
Cu catalysed direct fluorination (distilled) |
Yield [purity %] |
52% [n/a] |
77 [96%] |
99 [95%] (77 [99%+]) |
Atom economy (AE) |
39.1 |
39.0 |
89.9 |
Reaction mass efficiency (RME) |
20.6 |
33.0 |
88.6 (69.3) |
Step MI/PMI total |
62.1 |
6.9 |
9.0 (11.6) |
PMI/MI reaction |
18.0 |
5.5 |
4.6 (6.1) |
PMI/MI solvents |
48.8 |
3.3 |
6.7 (8.6) |
PMI/MI workup |
44.1 |
3.3 |
4.3 (5.5) |
Solvents |
MeOH |
|
Xylenes |
|
Acetonitrile |
|
Et2O |
|
|
Ethyl–acetate |
|
Catalyst |
— |
|
— |
|
Cu(NO3)2·2.5H2O |
|
Critical element |
— |
|
— |
|
Cu (recoverable) |
|
Energy |
High |
|
High |
|
Low (medium) |
|
|
Workup/purification |
Extraction |
|
Extraction |
|
Extraction |
|
Evaporation |
Multiple |
Evaporation |
|
Vac.-Distill. |
Vac.- Distill. |
(Vac. Distill.) |
|
Health & safety |
Corrosive |
|
Corrosive |
|
Corrosive |
|
Toxic |
Toxic |
Toxic |
Flammable |
|
Oxidiser |
Chemicals of environmental concern |
— |
— |
|
|
Availability |
Expensive |
|
Good |
|
Good |
|
The first obvious advantage of the direct fluorination process is that diethyl fluoromalonate is obtained in one synthetic step in excellent yield and no purification is necessary to achieve sufficiently pure material for subsequent synthetic steps (99% yield, 95% purity). The yields of the HFP and Halex methods are significantly lower and vacuum distillation processes are reported to be used to obtain the desired purity. The atom economy and reaction mass efficiency of the direct fluorination reaction are significantly higher than those of the competing synthetic processes mostly because fluorination only requires a single synthetic step and the only significant side product is one equivalent of HF. The low atom economy of the halogen exchange is explained by the high molecular weight reagents used for both the chlorination (SO2Cl2) and the halogen exchange (Et3N·3HF) steps. The PMI metrics indicate how much waste is generated during the production of 1 kg of product, and solvents are key contributors. However, for the direct fluorination process, solvents used in the reaction (MeCN) and work-up procedure (EtOAc) may be recovered and reused with no change in product profile.
As described in the patent literature, Bayer's halogen exchange reaction24 is a very efficient process, the reaction uses only a minimum amount of solvent and all process metrics look very promising apart from low atom economy due to the use of Et3N·3HF. A possible disadvantage of the halogen exchange process is the reported possibility of several time consuming vacuum distillation procedures, but this may not be necessary on the manufacturing scale.
Conclusion
Selective direct fluorination (SDF) process for the synthesis of 2-fluoromalonate esters have been optimised on a reasonable scale in the laboratory (40 g scale) both in terms of product yield and purity (99% crude yield, 95% purity, after distillation 77% yield and 99% purity) and green reaction metrics. The PMI value of the SDF process is, even at this relatively small scale, under 10, a benchmark figure that demonstrates an efficient and effective, environmentally benign chemical syntheses. A comparison of green metrics between the SDF process and established multi-step syntheses derived from hexafluoropropene ethanolysis and halogen exchange chemistry shows that SDF compares very favourably in terms of environmental impact for the synthesis of important fluorinated building blocks on larger scale.
Experimental
General
Proton, fluorine and carbon nuclear magnetic resonance spectra (1H, 19F and 13C NMR) were obtained using a Bruker 400 Ultrashield spectrometer (1H NMR at 400 MHz, 19F NMR at 376 MHz and 13C NMR at 101 MHz) using residual solvent peaks as the internal standard (1H NMR; CHCl3 at 7.26 ppm, 19F NMR; CFCl3 at 0.00 ppm and 13C NMR; CDCl3 at 77.16 ppm). NMR spectroscopic data are reported as follows: chemical shift (ppm), integration, multiplicity (s = singlet, d = doublet, t = triplet, q = quartet, m = multiplet), coupling constant (Hz) and assignment.
GC-MS data were obtained using a Trace GC-MS device (Thermo-Finnigan Corporation) operating in electron impact ionization (EI) mode. Accurate mass analysis was performed on a Xevo QtoF mass spectrometer (Waters Ltd, UK) with an accurate solids analysis probe (ASAP). Melting point data were obtained using a Gallenkamp apparatus at atmospheric pressure and are uncorrected. Infra-red (IR) spectroscopy was performed on a Perkin Elmer 1600 Series FTIR with an ATR probe. In situ IR spectroscopy was performed using a Mettler Toledo React IR instrument equipped with a diamond probe.
Fluorinations were carried out in a glass fluorination reactor (100 mL, 250 mL or 500 mL) unless otherwise stated. The reactor was built from a standard glass bottle with GL 45 thread joint and a PTFE screw cap or a glass flange head, equipped with a gas inlet/outlet head built of Stainless Steel, PTFE and FEP Swagelok components as described in earlier publications.15
Vacuum distillations were carried out using FISCHER® micro SPALTROHR®-column MMS 255 with manual fraction collection.
Diethyl malonate fluorination: general reaction
Diethyl malonate (3.20 g, 20 mmol) and copper nitrate hydrate (Cu(NO3)2·2.5H2O; 0.46 g, 2 mmol) were dissolved in acetonitrile (17 mL) and placed into the fluorination reactor and the mixture was cooled to 0–5 °C. After purging the system with N2 for 5 minutes, fluorine gas (10% v/v in N2, 45 mL min−1, 22 mmol) was passed into the stirred mixture for 2 h. The reactor was purged with nitrogen for 10 minutes, the solvent was removed in vacuo and the residue partitioned between water (10 mL) and ethyl acetate (10 mL). The aqueous phase was extracted with ethyl acetate (10 mL) and the combined organic layers were washed with saturated brine (10 mL). After drying over sodium sulfate, the solvent was evaporated to leave diethyl 2-fluoromalonate (3.37 g, 94% yield, 93.5% purity) as a colourless liquid. IR (neat, cm−1): 2986, 1747, 1243, 1187, 1097, 1020; δH (CDCl3, 400 MHz) 1.31 (6H, t, 3JHH 7.2, CH3), 4.31 (4H, q, 3JHH 7.2, CH2), 5.26 (1H, d, 2JHF 48.3, CHF); δF (CDCl3, 376 MHz): – 195.58 (d, 2JHF 48.3, CH–F); δC (CDCl3, 100 MHz) 14.04 (CH3), 62.80 (CH2), 85.39 (d, 1JCF 198.2, C–F), 164.20 (d, 2JCF 24.1, C
O); m/z (EI+) 179 (5%, [M + H]+), 133 (44%, [M − OEt]+), 105 (49%, [M − COOEt]+), 78 (100%, [CH2FCOOH]+). Spectroscopic data were identical with those previously reported15 and side products were identified from their reported 19F NMR shifts.
Diethyl fluoromalonate large scale fluorination
Diethyl malonate (40.0 g, 0.25 mol) and copper nitrate hydrate (Cu(NO3)2·2.5H2O; 5.81 g, 25 mmol) were dissolved in acetonitrile (200 mL) and placed in 500 mL fluorination vessel, cooled to 0–5 °C and stirred at 650 rpm using an overhead stirrer. After purging the system with N2 for 5 minutes, fluorine gas (20% v/v in N2, 80 mL min−1, 265 mmol) was introduced into the mixture for 6 hours and 30 minutes. The reactor was purged with nitrogen for 10 minutes, the solvent removed in vacuo and the residue partitioned between water (50 mL) and ethyl acetate (50 mL). The aqueous phase was extracted once more with ethyl acetate (50 mL) and the combined organic layers were washed with saturated NaHCO3 (25 mL) and brine (20 mL). After drying over sodium sulfate, the solvent was evaporated to leave diethyl 2-fluoromalonate (44.4 g, 99% yield, 95% purity) as a light yellow, transparent liquid. This crude product was distilled to afford high purity fluoromalonate (34.7 g, 77% yield, 99%+ purity) as a colourless liquid, bp. 102–103 °C (18 mbar), (lit.: 110–112 °C, 29 mbar),23b spectroscopic data as above.
Dimethyl 2-fluoromalonate
Dimethyl malonate (19.8 g, 0.15 mol) and Cu(NO3)2·2.5H2O (3.50 g, 15 mmol) were dissolved in acetonitrile (85 mL), the mixture was cooled to 0–5 °C and stirred at 650 rpm using an overhead stirrer. After purging the system with N2 for 5 minutes, fluorine gas (20% v/v in N2, 50 mL min−1, 170 mmol) was introduced into the reaction mixture for 7 h. After purging with nitrogen for 20 minutes, the solvent was removed in vacuo and the residue was partitioned between water (30 mL) and ethyl acetate (20 mL). The aqueous phase was extracted with ethyl acetate (2 × 20 mL) and the combined organic layer was washed with saturated brine (20 mL). After drying over sodium sulphate, the solvent was evaporated under reduced pressure to give dimethyl 2-fluoromalonate (21.8 g, 97% yield, 95% purity) as a colourless oil; IR (neat, cm−1) 2962, 1748, 1438, 1250, 1206, 1112, 1016; δH (CDCl3, 400 MHz) 3.85 (6H, s, CH3), 5.31 (1H, d, 2JHF 48.0, CHF); δF (CDCl3, 376 MHz): −195.73 (d, 2JHF 48.0, CH–F); δC (CDCl3, 100 MHz) 53.48 (CH3), 85.19 (d, 1JCF 197.2, C–F), 164.39 (d, 2JCF 24.0, C
O); m/z (EI+) 150 (3%, [M]+), 119 (42%, [M − OMe]+), 91 (73%, [M − COOMe]+), 59 (100%, [COOMe]+). Spectroscopic data in agreement with previously published data.23b Crystallographic data has been deposited at the Cambridge Crystallographic Database centre as file CCDC 1050160.
Di-tert-butyl 2-fluoromalonate
Di-tert-butylmalonate (12.0 g, 55 mmol) and copper nitrate catalyst (1.16 g, 5 mmol) were dissolved in acetonitrile (50 mL), placed in a fluorination vessel and the mixture was cooled to 0–5 °C. After purging the system with N2 for 5 minutes, fluorine gas (20% v/v in N2, 44 mL min−1, 60 mmol) was introduced for 5 h. After purging with nitrogen for 10 minutes, the solvent was removed under reduced pressure and the residue was partitioned between water (10 mL) and ethyl acetate (25 mL). The aqueous phase was extracted with ethyl acetate (25 mL) then the combined organic layer was washed with saturated brine (10 mL). After drying over sodium sulphate, the solvent was evaporated under reduced pressure to leave di-tert-butyl 2-fluoromalonate (12.57 g, 96% yield, 97% purity) as a colourless liquid; IR (neat, cm−1) 2980, 1744, 1369, 1252, 1143; δH (CDCl3, 400 MHz) 1.49 (18H, s, CH3), 5.01 (1H, d, 2JHF 48.9, CHF); δF (CDCl3, 376 MHz): −193.79 (d, 2JHF 48.9, CH–F); δC (CDCl3, 100 MHz) 27.95 (CH3), 84.01 (C–CH3), 85.97 (d, 1JCF 196.5, C–F), 163.32 (d, 2JCF 24.5, C
O); m/z (EI+) 162 (20%, [M − OtBu + H]+), 57 (100%, [tBu]+).
5-Fluoro-4,6-dihydroxypyrimidine
Formamidine acetate (2.06 g, 20 mmol) was added to the solution of sodium (1.38 g, 60 mmol) in anhydrous ethanol (40 mL) and the mixture was heated to reflux. Diethyl 2-fluoromalonate (3.20 g, 18 mmol) was added dropwise over 20 minutes and the mixture was heated at reflux for 6 h. After cooling to room temperature, the solution was evaporated to dryness, the residue was dissolved in water (20 mL), acidified with HCl (5 mL), the precipitate was filtered, washed with water (5 mL), ethanol (2 × 5 mL) and diethyl ether (2 × 5 mL). After drying in vacuo, 5-fluoro-4,6-dihydroxypyrimidine (1.50 g, 64%) was obtained as a brown powder. m.p.: >300 °C; ([M + H]+, 131.0244, C4H4FN2O2 requires: [M]+, 131.0257); IR (neat, cm−1) 3053, 2639, 1633, 1547, 1388, 1213; δH (DMSO d6, 400 MHz) 7.90 (1H, s, C–H), 12.38 (2H, bs, OH); δF (DMSO d6, 376 MHz): – 178.06 (s); δC (DMSO d6, 100 MHz) 132.79 (d, 1JCF 235.6, C–F), 144.46 (d, 4JCF 7.7, C–H), 155.83 (d, 2JCF 15.0, C–OH); m/z (ASAP) 131 (100%, [M + H]+).21a
2-Amino-5-fluoro-4,6-dihydroxypyrimidine
Guanidine sulfate (5.95 g, 55 mmol) was added to the solution of sodium (2.50 g, 110 mmol) in anhydrous ethanol (100 mL) and the mixture was heated to reflux. Diethyl 2-fluoromalonate (8.90 g, 50 mmol, 93% pure) was added dropwise over 20 minutes and the mixture was heated at reflux for 6 h. After cooling to room temperature, the solution was evaporated to dryness, the residue was dissolved in water (20 mL), neutralised with HCl to pH 7, the precipitated product was filtered, washed with water (5 mL), ethanol (2 × 5 mL) and diethyl ether (2 × 5 mL). After drying in vacuo, 2-amino-5-fluoro-4,6-dihydroxypyrimidine (6.21 g, 86%) was obtained as a pink powder. m.p.: >300 °C ([M + H]+, 146.0357, C4H5FN3O2 requires: [M]+, 146.0366); IR (neat,cm−1) 3343, 3100, 2916, 2731, 1600, 1557, 1415, 1358, 1204; δH (DMSO d6, 400 MHz) 7.00 (2H, bs, N–H), 11.1 (2H, bs, OH); δF (DMSO d6, 376 MHz): – 197.05 (s); δC (DMSO d6, 100 MHz) 125.13 (d, 1JCF 208.6, C–F), 149.26 (d, 4JCF 2.3, C–H), 155.12 (d, 2JCF 18.0, C–OH); m/z (ASAP) 146 (100%, [M + H]+).18a
5-Fluorobarbituric acid
Urea (1.50 g, 25 mmol) was added to the solution of sodium (1.2 g, 53 mmol) in anhydrous ethanol (50 mL) and the mixture was heated to reflux. Diethyl 2-fluoromalonate (4.45 g, 25 mmol) was added dropwise over 10 minutes and the mixture was heated at reflux for 1 h. After cooling to room temperature, the solution was filtered, the residue was washed with ethanol (20 mL), dissolved in water (30 mL) and acidified with HCl to pH 1. The precipitated product was recrystallized from the liquor to afford 5-fluorobarbituric acid (1.87 g, 51%) as a tan powder. m.p.: >300 °C; ([M + H]+, 147.0206, C4H4FN2O3 requires: [M]+, 147.0204); IR (neat,cm−1) 2926, 2828, 1578, 1383, 1241, 1128; δF (D2O + NaOD, 376 MHz): – 191.95 (s); δC (D2O + NaOD, 100 MHz) 131.89 (d, 1JCF 214.4, C–F), 157.77 (d, 4JCF 6.2, C–NH), 164.59 (d, 2JCF 13.4, C
O); m/z (ASAP) 147 (25%, [M + H]+).18a
3-Fluoro-1-H-1,5-benzodiazepine-2,4-dione
o-Phenylenediamine (2.70 g, 25 mmol) was added to the solution of sodium (1.2 g, 53 mmol) in anhydrous ethanol (50 mL) and the mixture was heated to reflux. Diethyl 2-fluoromalonate (4.45 g, 25 mmol) was added dropwise over 10 minutes and the mixture was heated at reflux for 2 h. After cooling to room temperature, the solution was filtered, the residue was washed with ethanol (20 mL), dissolved in water (30 mL) and acidified with HCl to pH 1. The mixture was cooled in ice, filtered, washed with water (2 × 10mL) and dried in vacuo to afford 3-fluoro-1-H-1,5-benzodiazepine-2,4-dione (3.23 g, 68%) as a tan powder. m.p.: >300 °C, ([M + H]+, 195.0567, C9H8FN2O2 requires: [M]+, 195.0570); IR (neat,cm−1) 3084, 2951, 1727, 1681, 1500, 1159; δH (DMSO d6, 400 MHz): 5.57 (1H, d, 2JHF 46.4, CHF), 7.15–7.19 (2H, m, Ar–H), 7.22 (2H, dt, 3JHH 6.6, 4JHH 3.5, Ar–H), 10.81 (2H, bs N–H); δF (DMSO d6, 376 MHz): – 207.99 (d, 2JHF 46.4 C–F); δC (DMSO d6, 100 MHz) 85.12 (d, 1JCF 184.5, C–F), 122.55, 125.52, 128.41, 163.36 (d, 2JCF 23.2, C
O), 164.59 (d, 2JCF 13.4, C
O); m/z (ASAP) 195 (100%, [M + H]+), 135 (23%, [M − COCHF]+).23b
Acknowledgements
The research included in this publication received funding from the European Community's Seventh Framework Programme (FP7/2007–2013) and EFPIA companies’ in kind contribution for the Innovative Medicine Initiative under Grant Agreement no. 115360 (Chemical manufacturing methods for the 21st century pharmaceutical industries, CHEM21). We thank Dr D. S. Yufit for X-ray crystallographic analyses and Dr C. R. McElroy for useful discussions regarding the use of green metrics.
References
-
(a) K. Muller, C. Faeh and F. Diederich, Science, 2007, 317, 1881–1886 CrossRef PubMed;
(b)
Fluorine in Medicinal Chemistry and Chemical Biology, ed. I. Ojima, Wiley-Blackwell, Oxford, 2009 Search PubMed;
(c) S. Purser, P. R. Moore, S. Swallow and V. Gouverneur, Chem. Soc. Rev., 2008, 37, 320–330 RSC;
(d) C. Isanbor and D. O'Hagan, J. Fluorine Chem., 2006, 127, 303–319 CrossRef CAS PubMed;
(e) K. L. Kirk, J. Fluorine Chem., 2006, 127, 1013–1029 CrossRef CAS PubMed.
-
(a) E. A. Ilardi, E. Vitaku and J. T. Njardarson, J. Med. Chem., 2014, 57, 2832–2842 CrossRef CAS PubMed;
(b) B. R. Smith, C. M. Eastman and E. J. Njardarson, J. Med. Chem., 2014, 57, 9764–9773 CrossRef CAS PubMed;
(c) W. K. Hagmann, J. Med. Chem., 2008, 51, 4359–4369 CrossRef CAS PubMed;
(d) FDA Approved Drug Products http://www.accessdata.fda.gov/scripts/cder/drugsatfda/index.cfm?fuseaction=Reports.ReportsMenu (Accessed 20 January 2015).
-
(a)
B. Baasner, H. Hagemann and J. C. Tatlow, Houben-Weyl Organofluorine Compounds, Vol. E10a, Thieme, Stuttgart, 2000 Search PubMed;
(b)
R. D. Chambers, Fluorine in Organic Chemistry, Wiley-Blackwell, Oxford, 2004 Search PubMed;
(c)
K. Uneyama, Organofluorine Chemistry, Blackwell, Oxford, 2006 Search PubMed;
(d) T. Liang, C. N. Neumann and T. Ritter, Angew. Chem., Int. Ed., 2013, 52, 8214–8264 CrossRef CAS PubMed.
-
(a) K. Alfonsi, J. Colberg, P. J. Dunn, T. Fevig, S. Jennings, T. A. Johnson, H. P. Kleine, C. Knight, M. A. Nagy, D. A. Perry and M. Stefaniak, Green Chem., 2008, 10, 31–36 RSC;
(b) R. K. Henderson, C. Jimenez-Gonalez, D. J. C. Constable, S. R. Alston, G. G. A. Inglis, G. Fisher, J. Sherwood, S. P. Binksa and A. D. Curzons, Green Chem., 2011, 13, 854–862 RSC;
(c) D. Prat, O. Pardigon, H.-W. Flemming, S. Letestu, V. Ducandas, P. Isnard, E. Guntrum, T. Senac, S. Ruisseau, P. Cruciani and P. Hosek, Org. Process Res. Dev., 2013, 17, 1517–1521 CrossRef CAS;
(d) R. K. Henderson, A. P. Hill, A. M. Redman and H. F. Sneddon, Green Chem., 2015, 17, 945–949 RSC;
(e) D. Prat, J. Hayler and A. Wells, Green Chem., 2014, 16, 4546–4551 RSC.
-
(a) D. J. C. Constable, A. D. Curzons and V. L. Cunningham, Green Chem., 2002, 4, 521–527 RSC;
(b) J. Andraos, Org. Process Res. Dev., 2005, 9, 149–163 CrossRef CAS;
(c) J. Augé, Green Chem., 2008, 10, 225–231 RSC;
(d) C. Jimenez-Gonzalez, C. S. Ponder, Q. B. Broxterman and J. B. Manley, Org. Process Res. Dev., 2011, 15, 912–917 CrossRef CAS;
(e)
Green Chemistry in the Pharmaceutical Industry, ed. P. J. Dunn, A. S. Wells and M. T. Williams, Wiley-VCH, Weinheim, 2010 Search PubMed.
-
(a) H. Suschitzky, Adv. Fluorine Chem., 1965, 4, 1–30 CAS;
(b)
G. Sandford, Fluoroarenes, in Science of Synthesis. Houben-Weyl Methods of Molecular Transformations. Vol. 31a: Compounds with Two Carbon-Heteroatom Bonds, ed. C. A. Ramsden, Thieme, Stuttgart, 2007, pp. 21–78 Search PubMed.
-
(a) R. Filler, Adv. Fluorine Chem., 1970, 6, 1–42 CAS;
(b)
G. Sandford, Trihalides, in Comprehensive Organic Functional Group Transformations 2, ed. A. R. Katritzky and C. J. Rees, Elsevier, Amsterdam, 2004, vol. 6, pp. 1–22 Search PubMed.
- G. Sandford, J. Fluorine Chem., 2007, 128, 90–104 CrossRef CAS PubMed.
-
(a)
S. A. Giller, A. Lazdinsh, A. K. Veinberg, A. Y. Sinker, I. L. Knunyants, L. S. German and N. B. Kazmina, US3846429, 1974 Search PubMed;
(b)
P. D. Schuman, P. Tarrant, D. A. Warner and G. Westmoreland, US3954758, 1976 Search PubMed;
(c)
T. Takahara and S. Misaki, US4082752, 1978 Search PubMed;
(d)
T. Umemoto and E. Ogura, US4631342, 1986 Search PubMed.
- M. Butters, J. Ebbs, S. P. Green, J. MacRare, M. C. Morland, C. W. Murtiashaw and A. J. Pettman, Org. Process Res. Dev., 2001, 5, 28–36 CrossRef CAS.
- J. F. Ellis and G. F. May, J. Fluorine Chem., 1986, 33, 133–147 CrossRef CAS.
- Linde on site fluorine generation cells: http://www.linde-gas.com/en/products_and_supply/electronic_gases_and_chemicals/on_site_gas_generation/generation_f_on_site_fluorine_generation/index.html (Accessed 20 January 2015).
-
(a) R. D. Chambers, M. P. Greenhall and J. Hutchinson, Tetrahedron, 1996, 52, 1–8 CrossRef CAS;
(b) R. D. Chambers, J. Hutchinson, A. S. Batsanov, C. W. Lehmann and D. Y. Naumov, J. Chem. Soc., Perkin Trans. 1, 1996, 2271–2275 RSC.
- R. D. Chambers, M. A. Fox and G. Sandford, Lab Chip, 2005, 5, 1132–1139 RSC.
-
(a) R. D. Chambers, J. Hutchinson and J. Thomson, J. Fluorine Chem., 1996, 78, 165–166 CrossRef CAS;
(b) R. D. Chambers, M. A. Fox, D. Holling, T. Nakano, T. Okazoe and G. Sandford, Chem. Eng. Technol., 2005, 28, 344–352 CrossRef CAS;
(c) R. D. Chambers and J. Hutchinson, J. Fluorine Chem., 1998, 92, 45–52 CrossRef CAS.
-
(a) J. Dubois, C. Foures, S. Bory, S. Falcou, M. Gaudry and A. Marquet, Tetrahedron, 1991, 47, 1001–1012 CrossRef CAS;
(b) F. L. M. Pattison, R. L. Buchanan and F. H. Dean, Can. J. Chem., 1965, 43, 1700–1713 CrossRef CAS;
(c) R. L. Buchanan and F. L. M. Pattison, Can. J. Chem., 1965, 43, 3466–3468 CrossRef CAS.
-
(a) T. Kawasaki, T. Ichige and T. Kitazume, J. Org. Chem., 1998, 63, 7525–7528 CrossRef CAS;
(b) D. Y. Kim, S. M. Kim, K. O. Koh, J. Y. Mang and K. Lee, Bull. Korean Chem. Soc., 2003, 24, 1425–1426 CrossRef CAS;
(c) X. Companyó, M. Hejnová, M. Kamlar, J. Vesely, A. Moyano and R. Rios, Tetrahedron Lett., 2009, 50, 5021–5024 CrossRef PubMed;
(d) S. H. Kang and D. Y. Kim, Bull. Korean Chem. Soc., 2009, 30, 1439–1440 CrossRef CAS;
(e) K. Kwon, S. M. Kim and D. Y. Kim, J. Fluorine Chem., 2009, 130, 759–761 CrossRef PubMed;
(f) H. Li, L. Zu, H. Xie and W. Wang, Synthesis, 2009, 1525–1530 CAS.
-
(a) E. D. Bergmann, S. Cohen and I. Dharak, J. Chem. Soc., 1959, 3286–3289 RSC;
(b) A. Harsanyi and G. Sandford, Beilstein J. Org. Chem., 2014, 10, 2013–2019 CrossRef PubMed;
(c) C. A. Fisher, A. Harsanyi, G. Sandford, D. S. Yufit and J. A. K. Howard, Chimia, 2014, 68, 425–429 CrossRef CAS PubMed.
- A. Harsanyi and G. Sandford, Org. Process Res. Dev., 2014, 18, 981–992 CrossRef CAS.
-
(a)
M. Hirotsuka, J. Sasaki, H. Kamiyama and M. Oshida, WO2009142184, 2009 Search PubMed;
(b)
G. Quin, B. Norton, X. Liao, A. N. Knox, Y. Fang, J. Lee, J. C. Dreabit, S. B. Christiensen, A. B. Benowitz and K. M. Aubart, WO2009061879, 2009 Search PubMed;
(c)
P. Eastwood, R. J. Gonzalez, T. J. Bach, S. L. M. Pages, M. J. Taltavull, J. J. F. Caturla and V. G. Matassa, WO2011076419, 2011 Search PubMed;
(d)
B. J. Branstetter, M. A. Letavic, K. S. Ly, D. A. Rudolph, B. M. Savall, C. R. Shah and B. T. Shireman, WO201105202, 2011 Search PubMed;
(e)
M. Brollo, A. Clauss, Y. El Ahmad, B. Filoche-Romme, F. Halley, K. A. Karlsson, G. Marciniak, B. Ronan, L. Schio, B. Vivet, F. Viviani and A. Zimmermann, WO2011001112, 2011 Search PubMed.
-
(a)
H. Weintritt, U. Stelzer, H. Gayer and W. Hubsch, US20030092723, 2003 Search PubMed;
(b) Bayer Annual Report 2010, p. 66 on http://www.bayer.com/en/annual-reports.aspx (Accessed 20 January 2015).
- Y. Zhao, L. Zhu, D. P. Provencal, T. A. Miller, C. O'Bryan, M. Langston, M. Shen, D. Bailey, D. Sha, T. Palmer, T. Ho and M. Li, Org. Process Res. Dev., 2012, 16, 1652–1659 CrossRef CAS.
-
(a) N. Ishikawa and A. Takaoka, Chem. Lett., 1981, 107–110 CrossRef CAS;
(b) N. Ishikawa, A. Takaoka and M. K. Ibrahim, J. Fluorine Chem., 1984, 25, 203–212 CrossRef CAS.
-
(a)
T. Müh, P. Fiedler, H. Weintritt, W. Westerkamp and A. Reinecke, WO2002016304, 2002 Search PubMed;
(b)
A. Günther, H. Weintritt and S. Böhm, WO2005019154, 2005 Search PubMed;
(c)
M. Braun and C. Brosch, WO2002050838, 2002 Search PubMed.
- ReactIR website: http://us.mt.com/us/en/home/products/L1_AutochemProducts/ReactIR.html (Accessed 20 January 2015).
- R. D. Chambers, A. M. Kenwright, M. Parsons, G. Sandford and J. S. Moilliet, J. Chem. Soc., Perkin Trans. 1, 2002, 2190–2197 RSC.
- R. D. Chambers, C. J. Skinner, J. Hutchinson and J. Thomson, J. Chem. Soc., Perkin Trans. 1, 1996, 605–609 RSC.
- C. R. McElroy, A. Constantinou, L. C. Jones, L. Summerton and J. H. Clark, Green Chem. Search PubMed , submitted.
- OECD High Production Volume Chemicals: http://webnet.oecd.org/hpv/ui/SponsoredSubstances.aspx (Accessed 20 January 2015).
- Z. Budesinsky, M. Budesinsky and A. Svab, Collect. Czech. Chem. Commun., 1981, 46, 2254–2262 CrossRef CAS.
Footnote |
† Electronic supplementary information (ESI) available: Green metrics calculations are contained within the supporting information file accompanying this publication. CCDC 1050160. For ESI and crystallographic data in CIF or other electronic format see DOI: 10.1039/c5gc00402k |
|
This journal is © The Royal Society of Chemistry 2015 |
Click here to see how this site uses Cookies. View our privacy policy here.