Research highlights: under-recognized precursors and sources for disinfection byproduct formation
First published on 18th June 2015
Disinfection byproducts (DBPs) are a significant concern because of their ubiquitous presence in finished water following disinfection and their toxicity at very low concentrations (ng–μg L−1).1 Trihalomethanes (THMs), haloacetic acids (HAA5), bromate, and chlorite have been regulated in drinking water by the U.S. Environmental Protection Agency (US EPA), and many others such as N-nitrosodimethylamine (NDMA) and chlorate are in the contaminant candidate list that are considered for regulation. Extensive studies have been conducted to understand toxicity, occurrence, formation, and effective control of DBPs. Natural organic matter (NOM) and naturally present bromide and iodide salts in source waters are believed to be the primary precursors for DBP formation via interactions with strong oxidative disinfectants.2 However, emerging DBPs and previously under-recognized precursors, sources, and formation pathways are still being identified. This Highlight article covers several unconventional precursors and sources for DBP formation from recent studies: hydraulic fracturing wastewater (HFW), N-functionalized carbon nanotubes, and nanofiltration membranes.
Hydraulic fracturing wastewater (HFW)-impacted drinking water supplies
While hydraulic fracturing has enabled and improved the production of natural gas from low permeability fossil fuel reservoirs over the past decade in the U.S., there are also concerns about adverse impacts on the environment. One such concern is that discharge of hydraulic fracturing wastewater increases concentrations of bromide and iodide, important DBP precursors, in receiving surface waters.1,2 One study shows that bromide concentrations in Pennsylvania surface waters near wastewater treatment plants receiving HFW increased from 72 (±81) μg L−1 (±1σ) to up to 300 μg L−1 because of increased drilling.3 Parker et al.4 evaluated the impacts of HFWs on the formation and speciation of DBPs during chlorination, chloramination, and ozonation of HFW blended freshwaters (Fig. 1). During chlorination, river water blended with only 0.01% HFW shifted the formation of DBPs towards brominated THMs, iodinated THMs, and brominated haloacetonitriles (HANs). Increases in HFW content to 0.03% further enhanced formation of all these compound classes. Chloramination of the blended waters led to the formation of iodinated THMs at lower pH values (<6). The highest NDMA formation during chloramination was observed for the water sample with the lowest bromide and highest iodide content. Bromide is thought to catalyze NDMA formation, but this study suggests that iodide could be a more potent catalyst. Conversely, chloramination reduced the formation of HANs and regulated THMs relative to chlorination. Ozonation of HFW-impacted river water promoted bromate formation. Bromate concentrations increased linearly with the fraction contribution of HFW, apparently due to the increasing bromide concentration, though factors other than bromide concentration (e.g., iodide, nitrite, and ammonium) can also influence bromate formation.
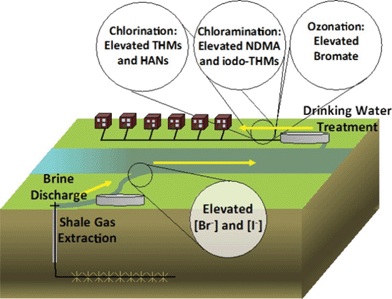 |
| Fig. 1 HFWs impacted formation and speciation of DBPs by chlorination, chloramination, and ozonation treatments. Reprinted with permission from ref. 4: K. M. Parker, T. Zeng, J. Harkness, A. Vengosh and W. A. Mitch, Environ. Sci. Technol., 2014, 48, 11161–11169. Copyright 2014 American Chemical Society. | |
N-Functionalized carbon nanotubes as a source and precursor of NDMA
Carbon nanotubes (CNTs) are emerging in industrial applications, and some of their unique properties are achieved through the introduction of diverse surface functional groups. CNTs may have unexpected impacts on the environment, and one potential impact is the generation of DBPs due to the presence and reactivity of CNTs during disinfection of drinking water. NDMA, the most widely detected nitrosamine DBP in drinking water, is primarily formed via reactions between chloramines and organic nitrogen-containing precursors.5 A recent study reported by Verdugo et al.6 showed that commercially available CNTs with nitrogen-containing surface groups (N-CNTs) can act as both a source and precursor of NDMA. N-CNTs leached up to 50 ng of NDMA per mg of N-CNTs in aqueous suspension, a surprisingly high amount. The leached NDMA is likely to be a byproduct resulting from N-functionalization of pristine CNTs. Moreover, N-CNTs were able to form NDMA during chlorination, chloramination, and ozonation even after extensive washing, with the NDMA yield (i.e., production per unit mass of disinfectant demand) comparable to that reported for NOM. For N-CNTs with different compositions, NDMA production did not increase proportionally to chlorine demand (Fig. 2) or surface nitrogen concentration. For a particular N-CNT, NDMA production generally scaled with chlorine consumption at lower Cl2 concentrations (i.e., 0.1–1.5 mg L−1); however the availability of reactive surface N-containing sites of N-CNTs likely limited NDMA formation in the presence of excess chlorine (i.e., >10 mg Cl2 per L). Similar trends were also observed for chloramination and ozonation. NDMA yield varied significantly by N-CNT type for all the disinfection processes studied.
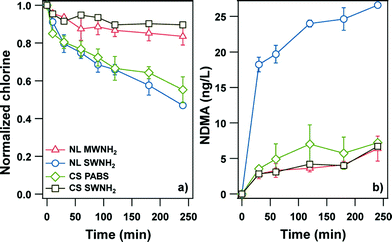 |
| Fig. 2 (a) Normalized chlorine consumption and (b) NDMA production during chlorination of N-CNT suspensions. NDMA concentrations were measured for 1 g L−1 N-CNT aqueous suspensions after sonication for 5 h. NL or CS represents CNTs acquired from NanoLab Inc. or Carbon Solutions, Inc. MWNH2 or SWNH2 represents multi-walled or single-walled carbon nanotubes functionalized with amide and/or amine groups, and PABS represents single-walled carbon nanotubes functionalized with N-containing polymer poly(m-aminobenzenesulfonic acid). Reprinted with permission from ref. 6: E. M. Verdugo, C. Krause, K. Genskow, Y. Han, J. Baltrusaitis, T. E. Mattes, R. L. Valentine and D. M. Cwiertny, Environ. Sci. Technol., 2014, 48, 9279–9287. Copyright 2014 American Chemical Society. | |
NDMA precursor release from nanofiltration membranes
Nanofiltration (NF) is a widely used water treatment process for removal of trace organic contaminants, viruses, dissolved organic matter, and multi-valent ions. NF membranes consist of polyamide composites, cellulose acetate blends, and polypiperazine amide together with a variety of additives and surface-coating substances, resulting in wide-ranging chemistries. High pressure is employed during NF, in which noncovalently bound chemicals are likely to be released from NF membranes and thus interact with disinfectants. Ersan et al.7 found that NDMA formation was observed in NF membrane permeate in the presence of chloramine for a broad range of virgin NF membranes (Fig. 3). This indicates that leaching of NDMA precursors may be a universal phenomenon for NF membranes. High pressure and flux were required to release tightly entrapped precursors, and leaching continued even after membranes had been washed with ~3900 L of distilled deionized water per square meter of membrane surface area.
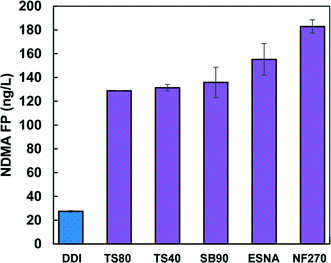 |
| Fig. 3 NDMA formation potentials of permeate from nanofiltration membranes for different manufacturers. NDMA FP represents NDMA formation potential, and its tests were conducted by reaction of membrane permeate with an excess chloramine solution (100 mg L−1 as Cl2) at pH 7.8 in the presence of a phosphate buffer and stored in the dark for 5 days for complete reactions of NDMA generation. TS40 is a polypiperazine amide based membrane, SB90 is a cellulose acetate based membrane, and TS80, ESNA, and NF270 are polyamide based membranes. Reprinted with permission from ref. 7: M. S. Ersan, D. A. Ladner and T. Karanfil, Environ. Sci. Technol. Lett., 2015, 2, 66–69. Copyright 2015 American Chemical Society. | |
In order to lower NDMA precursor leaching, acids, bases, and chlorine were used to flush and clean NF membranes. Though acids and bases are commonly applied to remove foulants from membranes, neither of them were able to effectively reduce the NDMA precursor leaching from the TS80 NF membrane. Chlorine is known to deactivate NDMA precursors, and cleaning of the TS80 and TS40 NF membranes with 1 mg L−1 of chlorine containing distilled deionized (DDI) water effectively reduced NDMA formation in the permeate after reaction with chloramine. Notably, although NDMA precursor leaching at the startup period of an NF process may be significant (NDMA formation of ~50–550 ng L−1), long-term use of the NF membranes will likely wash out the available precursors in practical applications.
Outlook
These studies indicate that under-recognized natural or anthropogenic chemicals/materials in water and wastewater treatment may act as unconventional precursors and sources for DBP formation. HFW potentially introduces bromide and iodide into the surface water for potable use, and it is able to form bromo- and iodo-DBPs, species typically more toxic than their chlorinated analogs. N-CNTs with unique chemical properties, which are likely to be widely applied in industry and may be subsequently released to the environment, not only leach NDMA but also promote formation of NDMA during disinfection. NF, a widely used water treatment process, can also be a source of NDMA contamination because of noncovalently bound NDMA precursors in membranes. Due to rapid growth in hydraulic fracturing, nanotechnology, and membrane-based processes, as well as the adverse health impacts associated with DBPs, it is necessary to consider the presence and associated risks of these unconventional precursors and sources for DBPs in water and wastewater treatment.
References
- S. D. Richardson and T. A. Ternes, Anal. Chem., 2014, 86, 2813–2848 CrossRef CAS PubMed.
-
S. D. Richardson and C. Postigo, in Emerging Organic Contaminants and Human Health, Springer, 2012, pp. 93–137 Search PubMed.
- R. D. Vidic, S. L. Brantley, J. M. Vandenbossche, D. Yoxtheimer and J. D. Abad, Science, 2013, 340, 1235009 CrossRef CAS PubMed.
- K. M. Parker, T. Zeng, J. Harkness, A. Vengosh and W. A. Mitch, Environ. Sci. Technol., 2014, 48, 11161–11169 CrossRef CAS PubMed.
- S. W. Krasner, W. A. Mitch, D. L. McCurry, D. Hanigan and P. Westerhoff, Water Res., 2013, 47, 4433–4450 CrossRef CAS PubMed.
- E. M. Verdugo, C. Krause, K. Genskow, Y. Han, J. Baltrusaitis, T. E. Mattes, R. L. Valentine and D. M. Cwiertny, Environ. Sci. Technol., 2014, 48, 9279–9287 CrossRef CAS PubMed.
- M. S. Ersan, D. A. Ladner and T. Karanfil, Environ. Sci. Technol. Lett., 2015, 2, 66–69 CrossRef CAS.
|
This journal is © The Royal Society of Chemistry 2015 |
Click here to see how this site uses Cookies. View our privacy policy here.