Research strategy to determine when novel nanohybrids pose unique environmental risks
Received
15th June 2014
, Accepted 26th November 2014
First published on 27th November 2014
Abstract
The production and use of increasingly complex hierarchical multifunctional ensembles of nanomaterials introduces emergent properties that will likely lead to uncertainty in the environmental health and safety (EHS) evaluation of nanohybrids (NHs). This perspective proposes principles to identify NHs with novel properties relevant to nano EHS research, and discusses specific challenges for EHS research on these materials. We propose a strategy for focusing nano EHS research efforts on relevant NH systems.
Nano impact
This perspective proposes principles to identify a nanohybrid (NH) for nano-EHS purposes, and highlights how emergent properties of NHs, i.e., properties different than the sum of the components, can influence assessment of their environmental risks. Though the overall framework of nano-EHS research, i.e., hazard identification, evaluation of fate, transport, transformation, and toxicity, exposure prediction, and risk assessment, will still apply, new strategies to narrow down the ever expanding space of conjugated materials are proposed.
|
Introduction
Recent focus on material synthesis and development at the nano-scale shows a clear shift from single material processing toward hierarchical assemblages. Even though synthesis of nanohybrid (NH) materials has been reported for over fifteen years (e.g., CdSe/ZnS core–shell QDs),1 only recently has there been an exponential growth in reports of more complex NHs and their incorporation into products,2 necessitating serious consideration of likely environmental impacts. Nanohybrids are combinations of more than one nanomaterial (NM) conjugated by strong molecular or macromolecular links or other physicochemical forces or are NMs modified by hard or soft coatings of unique chemical origin.3–5 The goal of such coupling of materials is to enhance existing functionality or to achieve multifunctionality. Such conjugations can occur between carbonaceous (e.g. fullerenes, carbon nanotubes, graphene) or metallic NMs (e.g., gold, silver, platinum, titania, zinc oxide) with or without complex organic coatings (porphyrins, pthalocyanines, enzymes).6–8 A wide array of applications, prominently energy and electronics, biomedical, catalysis, sensing, and environmental remediation, utilize these multifunctional NHs.3,6–10 Given the wide applicability and potential for unlimited combinations of NMs, the NHs are an ever-expanding set of novel materials. Better understanding of the risks of simpler NMs such as TiO2, Ag, or ZnO NMs is incomplete but improving.11 However, it is unclear if our nascent knowledge of the environmental risks of singular NMs (e.g., ZnO nanoparticles or carbon nanotubes) can also apply to a NH comprised of more than one of these components. The uncertainty in environmental health and safety (EHS) evaluation of this ensemble material class stems from the altered physicochemical properties of NHs and from emergence of novel properties that are not typically manifested by the component NMs individually. Thus, it is prudent to determine if and under what circumstances NHs, as a horizon class of nanomaterial, pose new and important environmental risks due to their altered or emergent properties. Here we provide a perspective on the issue of NH EHS research and propose a research strategy for focusing efforts on relevant systems to evaluate.
Principles to discern nanohybrids
Three principles are put forward to facilitate the identification of NHs (Fig. 1). (i) NHs are strongly conjugated or hybridized units, composed from two or more NMs, each with unique elemental composition or engineered crystalline phases. The strong linkage requirement limits NHs to materials that are expected to maintain their linkage for their intended application and even after their release to the environment. This principle would include core–shell nanoparticles composed of different metals, NMs with strongly-linked or -bonded organic coatings, carbon–metal conjugates (e.g., graphene–Pd,12 CdSe–pyridine–graphene13 (Fig. 1a), carbon nanotube-titania14), and metal–metal core–shell particles (e.g., quantum dots,1 iron oxide with silica coatings15 or titanium dioxide16 shells). Other example NHs in this category include heterocyclic porphyrin coated fullerenes17 or fluorophore modified gold NMs18 or functionalized gold NMs where ligands are bound with thiol linkages19 and NMs with grafted complex heterocyclic molecules (porphyrin,17,20 cyclodextrine21), proteins/enzymes, and similar biomolecules (cytochrome P450,22 amino acids, e.g., tyrosine23), metallocenes (ferrocene24), and hierarchical25,26 or layered27,28 structures. This principle would exclude metallic particles with an oxidized or sulfidized shell of the same metal (e.g., zero-valent iron with iron oxide shells,29 silver nanoparticle with sulfidized exterior30), NMs with weakly physisorbed surfactants or low molecular weight polymers (citrate coated nanogold or poly vinyl alcohol coated silver) or natural organic matter coated surfaces. (ii) NHs are materials where the hybridization leads to unique properties and/or reactivity, i.e., the NH properties are different than the simple sum of the components' properties. For example, when nano-TiO2 is strongly conjugated via Ti–O–C chemical bonds on carbon nanotube (CNT) external surfaces forming TiO2–CNT NHs the band gap of the NH is lower than TiO2 alone. This makes the NH photocatalytic under visible-light irradiation, whereas, the component materials are photoactive only in UV region.31 Similarly, conjugation of cytochrome P450 with CdSe quantum dots enable the cytochrome to become activated by the light induced reactive oxygen species (ROS, e.g., superoxides or hydroxy radicals) which otherwise was not possible (Fig. 1b).22 (iii) NHs are materials where the hybridization results in a change of dimensionality for at least one of the components. Examples of such NHs include ensembles of zero-dimensional fullerene and one-dimensional CNTs that result in unique three-dimensional nanobuds32 (Fig. 1c) when conjugated externally or nanotube-like one-dimensional nano-peapods33 when hybridized endohedrally. It should be noted that a NH must always satisfy the first principle, and will manifest properties captured in either principle (ii) or (iii), or both. These three principles will facilitate identification of NHs for nano-EHS evaluation. However, new hybridization schemes are likely to be discovered and applied, which might require modification or amendment of the stated principles.
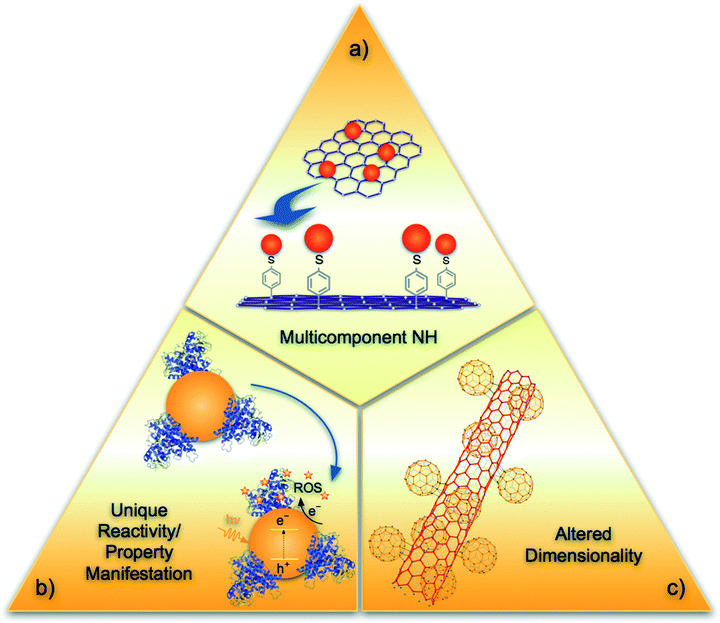 |
| Fig. 1 Schematic capturing NH discerning principles. (a) Multi-component aspect of NHs (e.g., CdSe–pyridine–graphene13), (b) unique reactivity/property manifestation (e.g., CdS quantum dots with enzyme cytochrome P450 can provide enzyme activation by light induced ROS22), and (c) altered dimensionality, an emerging property (e.g., nanobuds32). | |
Emergent properties and possible environmental interactions of nanohybrids
The key underlying rationale behind hybridization of NMs is to enhance existing functionality by changing the material properties. Hybridization therefore results in alterations of the component NMs' inherent physicochemical and electronic properties, where the altered property may not be realized by the sum of the individual components. Such novel properties are termed here as ‘emergent’ properties. Reported prominent alterations in physicochemical properties that are relevant to environmental fate and toxicity include changes in van der Waals and electrostatic interactions as well as dissolution and surface binding potential of conjugated ensembles. Fullerenes, when hybridized with single-walled carbon nanotubes (SWNTs) endohedrally, i.e., fullerenes inserted inside SWNTs (nano-peapods33), have van der Waals forces that are different than SWNTs or fullerene. This would change the aggregation of nano-peapods relative to SWNTs or fullerenes alone, potentially impacting the distribution of these materials in the environment (Fig. 2). Similarly, differences in the mode of conjugation, e.g., covalent vs. non-covalent attachments, alter dispersibility of nano-buds (exohedral SWNT-fullerene conjugates) in organic and aqueous media.34,35 Moreover, endohedral conjugations of fullerenes to SWNTs yield significantly different physical properties (differences in stiffness, morphology, and surface potential) than exohedral conjugations of these materials, which might also lead to different transport behavior despite being comprised of the same two types of NMs. Thus, even though both nano-peapods and nano-buds are composed of the same two component NMs (i.e., fullerenes and SWNTs), predicting their NH behavior from their component properties will not be possible because the mode of conjugation affects the properties that impact environmental fate including aggregation and deposition (Fig. 2).
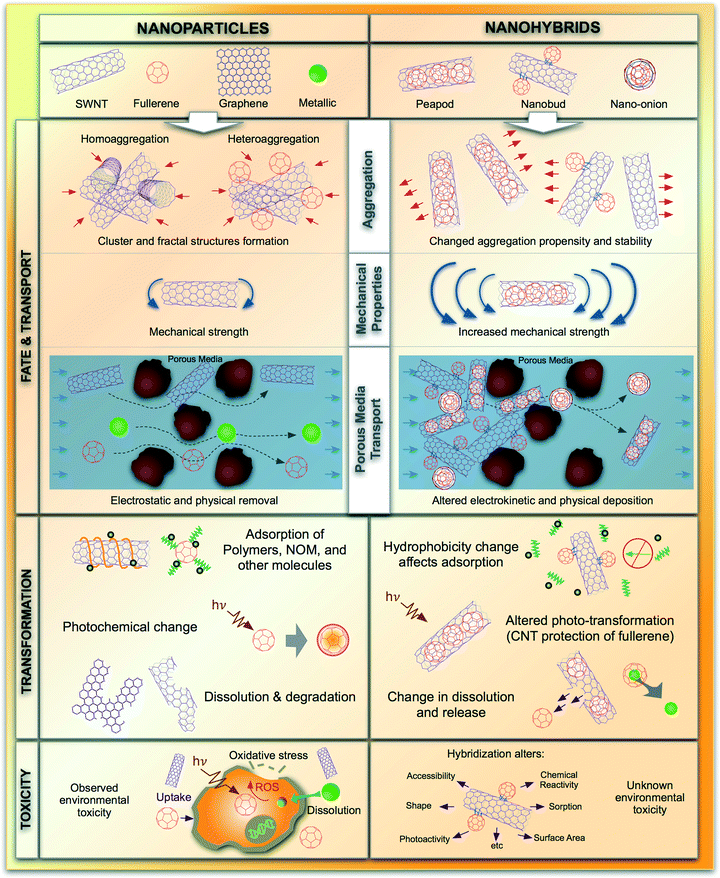 |
| Fig. 2 Schematic showing potential alteration of nano-EHS relevant properties of example NHs (right) in contrast to the properties of their component NMs (left). The fate and transport box shows how aggregation and filtration of SWNTs might be altered by hybridization and resulting alterations in electrostatics, stiffness, and dimensionality. The transformation box depicts how hybridization may alter adsorption, dissolution, and phototransformation. The toxicity box captures NH reactivity, photoactivity, and dimensional changes and the potential impact of these properties on cellular interactions and toxicity. | |
Another relevant example, where hybridization of NMs makes it difficult to assess the toxicity of NHs based on the component materials, is the use of layered coatings. For example, the presence of an amorphous nano-scale layer of silicon-dioxide (silica) on ZnO NPs decreased dissolution of the ZnO relative to uncoated ZnO NPs, without changing their optical properties, and significantly reduced DNA damage-potential compared to uncoated ZnO NMs of the same size.36 This principle can be applicable to component NMs and NHs alike. The process of hybridization of NMs to decrease potentially harmful effects, known as ‘safer by design’, is principled on the ability of NHs to have different properties than the component materials.37
Electronic properties of the NMs, known to control reactive oxygen species (ROS) generation and thereby mediate toxicity (Fig. 2), will also be altered via conjugation or overcoating. Attachment of multiple NMs modifies the energy states of the surface atoms and shifts or bends the inherent electron bands. For example, a recent study shows enhanced microbial toxicity of ZnO–graphene NHs due to increased ROS production;38 which likely have been caused by ZnO conduction band bending39 and subsequent overlap with the cellular redox potential.40 Environmental transformation processes relevant to NM fate, transport, and toxicity41 may undergo significant alterations with emergent NHs. For example, as mentioned previously, photoactivity and ROS generation potential of TiO2 changes from the ultra-violet (UV) to the visible range of illumination, when hybridized with CNTs;31 thus will likely have significant implications for photo-induced environmental transformation and toxicity.
Besides property modulations, NHs manifest novel properties that are unique to the parent NM properties. For example, hybridization among different NMs brings forth unique dimensionality; e.g., seamless conjugation of 0-D fullerenes onto 1-D SWNT surfaces, generates novel 3-D configurations of nano-buds.34 Such conjoined structures provide much greater, and perhaps unique, extended surface area for bio- and geo-macromolecule adsorption and altered cellular interaction. In addition, the extruding fullerene molecules, when functionalized, might induce physical repulsion in aqueous dispersions to enhance colloidal stability. Hybridization can also lead to very stiff materials with relatively unexplored biological effects.42 When fullerenes are endohedrally placed to punctuate a certain length of SWNTs, the nano-peapods' bending strength is found to increase significantly.43 Such enhancement in SWNT physical stiffness could affect their toxicity and their aggregation and deposition propensity by introducing a strong physical interaction.
Strategies and challenges for nanohybrid environmental implication studies
Even though NHs will likely present emergent properties that may challenge our understanding of nano-EHS, based on studies with single component systems and simple adsorbed layers, the overall framework for risk evaluation, i.e., identification and quantitation of NM release, properties and processes impacting nano-EHS (e.g. aggregation, transport, transformation), NM interaction with subcellular entities to ecosystems that are relevant to risk assessment, and organized knowledge and rapid feedback based research systems, remains a valid strategy.44–46 Within this framework the guiding principle remains to determine the influencing property or properties that will dictate NH behavior in release, fate and transport, exposure, and toxicity. However, when such properties are the result of conjugation or hybridization, the possible combinations of multiple materials are extremely large and go beyond the challenges around NP size, shape, and coatings type that are currently being addressed systematically by the nano-EHS community. Strategies are needed to rationally narrow down this ever-expanding space (Fig. 3), so that comprehensive nano-EHS evaluation can be performed with reliability and in a timely manner.
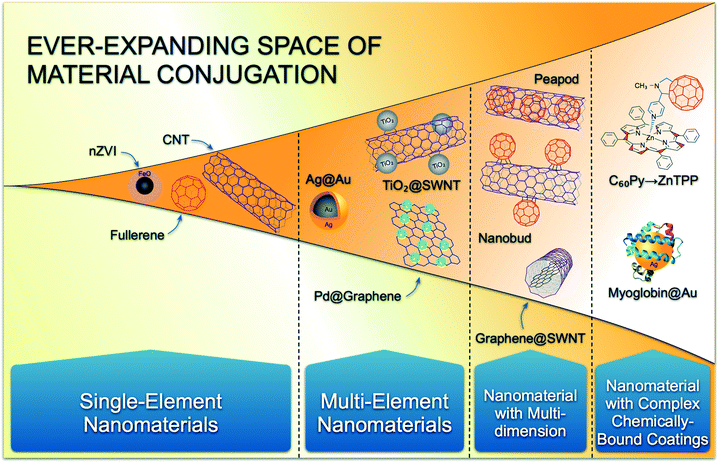 |
| Fig. 3 Schematic showing the ever-expanding space of nanomaterial conjugation and the resulting permutations of nanomaterials. | |
Overall, a strategy to address the nano-EHS implications of NHs will need to determine 1) if and when emergent properties arise, 2) the stability (integrity) of the NH under environmental conditions; i.e., whether a conjugated ensemble will remain and act as one unit during environmental exposure and transformation or will relatively quickly disintegrate into its component materials, and 3) which of these emergent properties poses unwanted environmental effects. The ability to characterize NHs and to measure the properties of these materials in biological and environmental matrices is essential to this effort. Clearly, NHs with emergent properties that can pose significant environmental risk—e.g., NHs with higher stiffness causing increased physical interaction or bent band gap resulting in higher propensity to produce ROS and higher resulting toxicity—and those that are likely to retain their hybridized identity in the environment will have higher likelihood to possess and maintain altered or emergent properties relative to the components.
Identifying and characterizing important emergent NH properties
Conjugation gives rise to emergent properties (discussed earlier), that may pose new risks compared to NMs already studied. However, the role of such property modulation in fate, transport, transformation, and toxicity is not well understood. Since it is difficult a priori to predict how a novel property will affect fate and toxicity, NHs with properties known to be risky (based on work with simpler NMs) should be given priority. For example, graphene nano-sheets when decorated with ZnO NMs increase effective surface area, where the graphene serves to sequester Zn2+ ions and facilitates NH-bacterial interaction;47 rough edges from 2-D graphene can physically disrupt cell membranes.48 It is acknowledged that environmentally emergent properties discussed herein are not exhaustive, rather are subject to future discovery by systematic research from the EHS community.
Due to hybridization, changes in dimensionality, electronic and optical signatures, dissolution and morphology occur, which need to be characterized accurately. As such new and unknown properties emerge from conjugation, modification of existing characterization techniques or adaptation of new ones will be necessary. A strategy can be to leverage existing characterization techniques first and measure the emergent properties that the technique is capable of measuring. For example, high resolution transmission electron microscopy may provide information regarding morphological changes.49 Elemental mapping utilizing scanning electron tunneling microscopy (STEM), X-ray spectroscopy, or dark field imaging with spectroscopy can be used to determine material distribution for these multi-element NHs.14,50 A second strategy can be to adopt or develop new techniques to measure emergent properties. For example, emergent mechanical properties, such as stiffness, can be measured using modified atomic force microscopy protocols51 or by nano-indentation.52,53 Complex and hierarchical coating morphology can be characterized utilizing STEM54 or neutron scattering.55,56 Such strategies will evolve, as the nano-EHS community identify and prioritize the emergent properties of NHs.
Characterizing NH stability in environmental and biological matrices
Central to nano-EHS evaluation of NHs is the stability (integrity) of the ensemble material during environmental transport, transformation, and exposure. NHs that maintain their unique properties in environmental and biological media could have unique, yet to be studied, EHS implications; so the stability of these NHs under environmentally relevant conditions needs to be evaluated. Characterization techniques to determine stability of the NHs in environmental and biological matrices are needed. For example, traditional spectroscopy techniques can be used to look for unique responses from conjugates. Ultra-violate visible (UV-Vis) and Raman spectroscopic signature of nanopeapods, filled with C60s or C70s, were shown to be unique compared to component NMs, which allowed an evaluation of the van der Waals interaction between the nanopeapods for these two fullerene classes.33 Radio-labeling one or both of the NMs can lead to exposure and stability evaluation.57,58 Also, novel physical separation and electron imaging based novel techniques need to be established to characterize stability of these conjugates under environmentally relevant conditions. In the longer term, more complete analysis of these emergent properties will likely require development of new methods for characterization.
Overcoming problems with heterogeneity of NHs
One important challenge for the nano-EHS community is characterizing heterogeneity within a particular batch of NMs, or between batches of NMs. This heterogeneity between NM batches makes it challenging to generalize nano-EHS results across studies.59,60 Heterogeneities can include, for example, differences in particle size distribution, coating mass or conformation, or chemical composition between or within batches.59,61 In addition, a rather homogeneous NM sample can become heterogeneous after environmental transformation; e.g., CNTs released in the environment may be partly photo-transformed, leading to a heterogeneous mixture of transformed and pristine CNT materials.62 Another example is the partial transformation of Ag NPs in soils or sediments,63 or ZnO NPs during wastewater treatment.64 This leads to Ag(0)/Ag2S core–shell particles in the case of Ag, or a mixture of various Zn-based particles for ZnO in a WWTP, each having different properties than the initial NM. This sample heterogeneity can affect behaviors of NMs in environmental and biological media.65 As NHs are conjugations of different NMs, the problem of heterogeneity between samples might be exacerbated compared to simpler NMs. One strategy to address this is to improve control of material properties during NH synthesis. Reproducible evaluations of NHs will require the availability of standard or reference materials.
Multi-element, heterocyclic and complex soft-layer coated, and conjugated NHs presenting emergent properties are already used in commerce. The health and safety evaluation of these materials is lacking. Identifying the EHS-relevant NHs, characterizing their integrity in soil and biological matrices, and development of techniques to measure their emergent properties are essential first steps for evaluating their environmental behavior. The proposed principles can help the nano-EHS community to narrow down the ever-expanding safety evaluation space of conjugated NH materials by focusing on materials with the most risky and long-lived novel properties.
References
- B. O. Dabbousi, J. Rodriguez-Viejo, F. V. Mikulec, J. R. Heine, H. Mattoussi, R. Ober, K. F. Jensen and M. G. Bawendi, J. Phys. Chem. B, 1997, 101, 9463–9475 CrossRef CAS.
- N. Aich, J. Plazas-Tuttle, J. R. Lead and N. B. Saleh, Environ. Chem., 2014 Search PubMed , (in Press).
- U. Banin, Y. Ben-Shahar and K. Vinokurov, Chem. Mater., 2013, 26, 97–110 CrossRef.
- L. H. Liu, R. Metivier, S. F. Wang and H. Wang, J. Nanomater., 2012, 2012, 1–2 Search PubMed.
- N. Saleh, A. R. M. N. Afrooz, J. Bisesi, N. Aich, J. Plazas-Tuttle and T. Sabo-Attwood, Nanomaterials, 2014, 4, 372–407 CrossRef PubMed.
- M. Vizuete, M. Barrejon, M. J. Gomez-Escalonilla and F. Langa, Nanoscale, 2012, 4, 4370–4381 RSC.
- D. Eder, Chem. Rev., 2010, 110, 1348–1385 CrossRef CAS PubMed.
- N. C. Bigall, W. J. Parak and D. Dorfs, Nano Today, 2012, 7, 282–296 CrossRef CAS PubMed.
- H. X. Kong, Curr. Opin. Solid State Mater. Sci., 2013, 17, 31–37 CrossRef CAS PubMed.
- T.-D. Nguyen, Colloids Surf., B, 2013, 103, 326–344 CrossRef CAS PubMed.
-
Research Progress on Environmental, Health, and Safety Aspects of Engineered Nanomaterials, The National Academies Press, 2013 Search PubMed.
- Y. Li, X. Fan, J. Qi, J. Ji, S. Wang, G. Zhang and F. Zhang, Nano Res., 2010, 3, 429–437 CrossRef CAS.
- M.-H. Jung and M.-J. Chu, Nanoscale, 2014, 6, 9241–9249 RSC.
- W. A. Rigdon, J. J. Sightler, D. Larrabee, E. McPherson and X. Huang, ECS Trans., 2013, 50, 1681–1692 CrossRef PubMed.
- Y. Lu, Y. Yin, B. T. Mayers and Y. Xia, Nano Lett., 2002, 2, 183–186 CrossRef CAS.
- D. Beydoun and R. Amal, Mater. Sci. Eng., B, 2002, 94, 71–81 CrossRef.
- D. Balbinot, S. Atalick, D. M. Guldi, M. Hatzimarinaki, A. Hirsch and N. Jux, J. Phys. Chem. B, 2003, 107, 13273–13279 CrossRef CAS.
- T. Ming, L. Zhao, H. Chen, K. C. Woo, J. Wang and H.-Q. Lin, Nano Lett., 2011, 11, 2296–2303 CrossRef CAS PubMed.
- G. H. Woehrle, L. O. Brown and J. E. Hutchison, J. Am. Chem. Soc., 2005, 127, 2172–2183 CrossRef CAS PubMed.
- I. Banerjee, D. Mondal, J. Martin and R. S. Kane, Langmuir, 2010, 26, 17369–17374 CrossRef CAS PubMed.
- G. Zhu, P. Gai, L. Wu, J. Zhang, X. Zhang and J. Chen, Chem. – Asian J., 2012, 7, 732–737 CrossRef CAS PubMed.
- B. I. Ipe and C. M. Niemeyer, Angew. Chem., Int. Ed., 2006, 45, 504–507 CrossRef CAS PubMed.
- S. Li, G. Zhao and H. Chen, J. Dispersion Sci. Technol., 2005, 26, 429–433 CrossRef CAS PubMed.
- X. Yang, Y. Lu, Y. Ma, Y. Li, F. Du and Y. Chen, Chem. Phys. Lett., 2006, 420, 416–420 CrossRef CAS PubMed.
- T. Hasobe, S. Fukuzumi and P. V. Kamat, Electrochem. Soc. Interface, 2006, 15, 47–51 CAS.
- D. M. Guldi, H. Taieb, G. M. A. Rahman, N. Tagmatarchis and M. Prato, Adv. Mater., 2005, 17, 871–875 CrossRef CAS.
- J.-M. Oh, M. Park, S.-T. Kim, J.-Y. Jung, Y.-G. Kang and J.-H. Choy, J. Phys. Chem. Solids, 2006, 67, 1024–1027 CrossRef CAS PubMed.
- J. Y. Kim, S.-J. Choi, J.-M. Oh, T. Park and J.-H. Choy, J. Nanosci. Nanotechnol., 2007, 7, 3700–3705 CrossRef CAS PubMed.
- N. Saleh, K. Sirk, Y. Liu, T. Phenrat, B. Dufour, K. Matyjaszewski, R. D. Tilton and G. V. Lowry, Environ. Eng. Sci., 2007, 24, 45–57 CrossRef CAS.
- C. Levard, E. M. Hotze, G. V. Lowry and G. E. Brown Jr., Environ. Sci. Technol., 2012, 46, 6900–6914 CrossRef CAS PubMed.
- C.-H. Wu, C.-Y. Kuo and S.-T. Chen, Environ. Technol., 2013, 34, 2513–2519 CrossRef CAS PubMed.
- A. G. Nasibulin, P. V. Pikhitsa, H. Jiang, D. P. Brown, A. V. Krasheninnikov, A. S. Anisimov, P. Queipo, A. Moisala, D. Gonzalez, G. Lientschnig, A. Hassanien, S. D. Shandakov, G. Lolli, D. E. Resasco, M. Choi, D. Tomanek and E. I. Kauppinen, Nat. Nanotechnol., 2007, 2, 156–161 CrossRef CAS PubMed.
- A. G. Ryabenko, N. A. Kiselev, J. L. Hutchison, T. N. Moroz, S. S. Bukalov, L. A. Mikhalitsyn, R. O. Loutfy and A. P. Moravsky, Carbon, 2007, 45, 1492–1505 CrossRef CAS PubMed.
- A. G. Nasibulin, P. V. Pikhitsa, H. Jiang, D. P. Brown, A. V. Krasheninnikov, A. S. Anisimov, P. Queipo, A. Moisala, D. Gonzalez, G. Lientschnig, A. Hassanien, S. D. Shandakov, G. Lolli, D. E. Resasco, M. Choi, D. Tomanek and E. I. Kauppinen, Nat. Nanotechnol., 2007, 2, 156–161 CrossRef CAS PubMed.
- Y. F. Shen, A. G. Skirtach, T. Seki, S. Yagai, H. G. Li, H. Moehwald and T. Nakanishi, J. Am. Chem. Soc., 2010, 132, 8566–8568 CrossRef CAS PubMed.
- G. A. Sotiriou, C. Watson, K. M. Murdaugh, T. H. Darrah, G. Pyrgiotakis, A. Elder, J. D. Brain and P. Demokritou, Environ. Sci.: Nano, 2014, 1, 144–153 RSC.
- J. E. Hutchison, ACS Nano, 2008, 2, 395–402 CrossRef CAS PubMed.
- T. Kavitha, A. I. Gopalan, K.-P. Lee and S.-Y. Park, Carbon, 2012, 50, 2994–3000 CrossRef CAS PubMed.
- J. O. Hwang, D. H. Lee, J. Y. Kim, T. H. Han, B. H. Kim, M. Park, K. No and S. O. Kim, J. Mater. Chem., 2011, 21, 3432–3437 RSC.
- H. Zhang, Z. Ji, T. Xia, H. Meng, C. Low-Kam, R. Liu, S. Pokhrel, S. Lin, X. Wang, Y.-P. Liao, M. Wang, L. Li, R. Rallo, R. Damoiseaux, D. Telesca, L. Mädler, Y. Cohen, J. I. Zink and A. E. Nel, ACS Nano, 2012, 6, 4349–4368 CrossRef CAS PubMed.
- G. V. Lowry, K. B. Gregory, S. C. Apte and J. R. Lead, Environ. Sci. Technol., 2012, 46, 6893–6899 CrossRef CAS PubMed.
- R. Subbiah, S. Ramasundaram, P. Du, K. Hyojin, D. Sung, K. Park, N.-E. Lee, K. Yun and K. J. Choi, J. R. Soc., Interface, 2013, 10, DOI:10.1098/rsif.2013.0694.
- J. Zhu, Z. Y. Pan, Y. X. Wang, L. Zhou and Q. Jiang, Nanotechnology, 2007, 18, 275702, DOI:10.1088/0957-4484/18/27/275702.
- H. National Research Council. Committee to Develop a Research Strategy for Environmental and S. A. o. E. Nanomaterials, A Research Strategy for Environmental, Health, and Safety Aspects of Engineered Nanomaterials, National Academies Press, 2012 Search PubMed.
-
J. A. Morris, Nanomaterial research strategy, U.S. Environmental Protection Agency, Office of Research and Development, Washington, D.C., 2009 Search PubMed.
-
K. Savolainen, U. Backman, D. Brouwer, B. Fadeel, T. Fernandes, T. Kuhlbusch, R. Landsiedel, I. Lynch and L. Pylkkänen, Nanosafety in Europe 2015–2025: Towards safe and sustainable nanomaterials and nanotechnology innovations, Nanosafety Research Center, Finish Insitute of Occupational Health, Helsinki, Finland, 2013 Search PubMed.
- Y.-W. Wang, A. Cao, Y. Jiang, X. Zhang, J.-H. Liu, Y. Liu and H. Wang, ACS Appl. Mater. Interfaces, 2014, 6, 2791–2798 CAS.
- Y. Li, H. Yuan, A. von dem Bussche, M. Creighton, R. H. Hurt, A. B. Kane and H. Gao, Proc. Natl. Acad. Sci. U. S. A., 2013, 110, 12295–12300 CrossRef CAS PubMed.
- P. S. Kumar, M. Selvakumar, P. Bhagabati, B. Bharathi, S. Karuthapandian and S. Balakumar, RSC Adv., 2014, 4, 32977–32986 RSC.
- A. R. Badireddy, M. R. Wiesner and J. Liu, Environ. Sci. Technol., 2012, 46, 10081–10088 CrossRef CAS PubMed.
- J.-P. Salvetat, G. A. D. Briggs, J.-M. Bonard, R. R. Bacsa, A. J. Kulik, T. Stöckli, N. A. Burnham and L. Forró, Phys. Rev. Lett., 1999, 82, 944–947 CrossRef CAS.
- J. Liu, Y. Yuan and S. Bashir, Energies, 2013, 6, 6476–6486 CrossRef CAS PubMed.
- C. Lee, X. Wei, J. W. Kysar and J. Hone, Science, 2008, 321, 385–388 CrossRef CAS PubMed.
- M. van SchooneveldMatti, A. Gloter, O. Stephan, L. F. Zagonel, R. Koole, A. Meijerink, J. M. MulderWillem and M. F. de GrootFrank, Nat. Nanotechnol., 2010, 5, 538–544 CrossRef CAS PubMed.
- P. Akcora, H. Liu, S. K. Kumar, J. Moll, Y. Li, B. C. Benicewicz, L. S. Schadler, D. Acehan, A. Z. Panagiotopoulos, V. Pryamitsyn, V. Ganesan, J. Ilavsky, P. Thiyagarajan, R. H. Colby and J. F. Douglas, Nat. Mater., 2009, 8, 354–359 CrossRef CAS PubMed.
- M. J. A. Hore, J. Ford, K. Ohno, R. J. Composto and B. Hammouda, Macromolecules, 2013, 46, 9341–9348 CrossRef CAS.
- D. Kryza, J. Taleb, M. Janier, L. Marmuse, I. Miladi, P. Bonazza, C. D. Louis, P. Perriat, S. P. Roux, O. Tillement and C. Billotey, Bioconjugate Chem., 2011, 22, 1145–1152 CrossRef CAS PubMed.
- E. J. Petersen, Q. Huang and J. W. J. Weber, Environ. Sci. Technol., 2008, 42, 3090–3095 CrossRef CAS.
- J. C. Bonner, R. M. Silva, A. J. Taylor, J. M. Brown, S. C. Hilderbrand, V. Castranova, D. Porter, A. Elder, G. Oberdorster and J. R. Harkema, Environ. Health Perspect., 2013, 121, 676–682 CrossRef CAS PubMed.
- T. M. Scown, E. M. Santos, B. D. Johnston, B. Gaiser, M. Baalousha, S. Mitov, J. R. Lead, V. Stone, T. F. Fernandes, M. Jepson, R. van Aerle and C. R. Tyler, Toxicol. Sci., 2010, 115, 521–534 CrossRef CAS PubMed.
- R. França, X. F. Zhang, T. Veres, L. H. Yahia and E. Sacher, J. Colloid Interface Sci., 2013, 389, 292–297 CrossRef PubMed.
- J. L. Bitter, J. Yang, S. Beigzadeh Milani, C. T. Jafvert and D. H. Fairbrother, Environ. Sci.: Nano, 2014, 1, 324–337 RSC.
- G. V. Lowry, B. P. Espinasse, A. R. Badireddy, C. J. Richardson, B. C. Reinsch, L. D. Bryant, A. J. Bone, A. Deonarine, S. Chae, M. Therezien, B. P. Colman, H. Hsu-Kim, E. S. Bernhardt, C. W. Matson and M. R. Wiesner, Environ. Sci. Technol., 2012, 46, 7027–7036 CrossRef CAS PubMed.
- R. Ma, C. Levard, J. D. Judy, J. M. Unrine, M. Durenkamp, B. Martin, B. Jefferson and G. V. Lowry, Environ. Sci. Technol., 2013, 48, 104–112 CrossRef PubMed.
- R. Huang, R. P. Carney, F. Stellacci and B. L. T. Lau, Langmuir, 2013, 29, 11560–11566 CrossRef CAS PubMed.
|
This journal is © The Royal Society of Chemistry 2015 |