Land use as an explanatory factor for potential phosphorus loss risk, assessed by P indices and their governing parameters
Received
27th May 2015
, Accepted 2nd June 2015
First published on 7th July 2015
Abstract
The total level of phosphorus (P) and the distribution of P pools in the topsoil are significantly affected by the excessive application of mineral and organic fertilizers connected with intensive agriculture. This leads to an increased potential risk for P loss, and then contributes to freshwater eutrophication. Soil test P (STP), P sorption index (PSI) and degree of P saturation (DPS) are commonly applied as proxies for assessing the risk of P loss. Although conceptually based, the empirical relationships between these operationally defined proxies and the actual P flux exhibit large spatial variations. Herein, a comprehensive synoptic study and monitoring of soil has been conducted in a watershed in north-eastern China. A set of conventional indicators for soil P loss risk were measured along with the main P pools, P sorption indices, texture, organic matter, as well as Fe and Al oxides and other mineral compositions. Moreover, detailed soil P speciation was conducted using phosphorus nuclear magnetic resonance (31P NMR) spectroscopy. In addition, phosphatase activities in the soils were determined for each land use soil category. The results reflected that the soil content of total P, total inorganic P and STP increased significantly following the order of increasing management intensity. STP, being strongly coupled to the application of P fertilizers, was a strong explanatory factor for the spatial differences in DPS – both between and within different land uses. The dominant inorganic and organic P species in the soils were orthophosphate and monoester-P, respectively. Their contents were oppositely correlated with the degree of management influence, with the amount of orthophosphate positively related. Alkaline phosphomonoesterase (AlP) represented the highest activities among the four representative phosphatases, i.e. enzymes that hydrolyze organic P – releasing labile orthophosphate. Orchard soils were found to contain the highest levels of monoester P as well as high AlP activities. This indicates a strong capacity to produce labile orthophosphate. Our results suggest that the type of land use can be employed as a general explanatory factor for considering the potential high risk of loss of P. Regionalized P loss parameters will further improve the accuracy of risk assessment.
Environmental impact
Empirical relationships between soil chemical proxies for phosphorus (P) loss and the actual P flux, causing eutrophication, exhibit large spatial variations. A comprehensive study focusing on the physicochemical characteristics of soils has been conducted in a typical agricultural region in north-eastern China. The set of conventional indicators for the risk of P loss were measured along with soil P pools and minerals. Moreover, detailed soil P speciation and phosphatase activities were determined for each land use category. This study manages to provide a clear conceptually based and empirically verified ranking of land-use categories according to their importance as potential P leaching risk to surface waters, which is especially useful for environmental managers as land-use maps are readily available.
|
1 Introduction
Enhanced P fluxes from terrestrial to aquatic systems have been identified as one of the most dominant causes for freshwater eutrophication due to the P-limited nature of aquatic algae and cyanobacteria.1,2 The main P source in agricultural regions is usually the diffusive drainage from farmland. This is especially the case in developed countries where implemented abatement actions have efficiently removed main P point sources.3,4
Accumulation of excess P in the top horizon of agricultural soils generally increases the risk for P loss to the aquatic ecosystem.1,5 Different land use categories differ significantly in the intensities of agricultural management practices, especially in regard to the application of inorganic and organic P fertilizers.6 Land use maps can therefore potentially be used to spatially distinguish between areas with different levels of total soil P as well as the relative sizes of P pools, and thereby areas with similar potential risk for P loss.
During the last decade, a number of soil P parameters, such as soil test P (STP), P sorption index (PSI) and the degree of P saturation (DPS), have been used as proxies for assessing the potential risk for P losses from soils to surface water.2 Several analytical studies have therefore been conducted to develop a suitable proxy method as a measure of both bioavailable P as well as to predict loss of dissolved reactive phosphorus (DRP, i.e. mainly orthophosphate). To date, Mehlich 3 P (M3P), Olsen P, and Bray-1 P are generally considered as the most accepted methods to determine the bioavailable pool of P (STP) in the soils. Among these, M3P has been most widely applied due to its applicability for both acidic and alkaline soils.7 DPS is the pool of STP relative to the capacity of soils to retain P. Some studies have found that this proxy is better than STP at predicting concentrations of DRP in runoff from the soils.8,9
A shortcoming when assessing the STP parameter is the lack of consideration of the main P containing compounds in soils. A number of studies have therefore been conducted in order to characterize and identify major soil P species using a variety of advanced analytical instrumentation.10 The employment of phosphorus NMR spectroscopy has greatly improved the understanding of soil P species, particularly the organic P forms quantified in relative terms. Moreover, this method is devoid of complex cleanup procedures and chromatographic separations thereby avoiding changes in the speciation during sample preparation.11 In addition, aluminum and iron oxides in the soils, which are generally considered as the most important soil phosphate adsorbents, need to be considered specifically in order to understand the mechanism of soil P sorption. The oxides adsorb phosphate by a substitution reaction where singly coordinated hydroxyl groups on the oxide surface (adsorption sites) are replaced by phosphate.12,13
Another important soil factor governing STP is the activity of phosphatase enzymes, as they play a central role in hydrolyzing soil organic P as well as condensed inorganic P forms.6 This cleavage of phosphate ester (C–O–P), phosphoanhydride (P–O–P) or phosphonate (C–P) bonds by the addition of water is predominantly mediated through the action of phosphatase enzymes.14 The product of these reactions is bioavailable orthophosphate. Knowledge of both soil P speciation and phosphatase activities is therefore needed in order to better understand and predict soil STP, and thus the potential environmental impact assessment.
In this study we rationalize for the employment of the spatially available information regarding land use as a combined proxy to assess the potential risk for soil phosphorus loss from a region. This is done by considering similarities within and consistent differences among the land use categories in regard to Fe and Al oxides, P pools, P sorption indices, soil P species and phosphatase activities. This approach is especially sought for in regions with intensive agricultural farming on soil with low P sorption capacity. An additional objective of this study is to identify the effects of different land use on the potential risk for loss of soil P.
2 Methods
2.1 Site description
The studied area is the local catchment of the large drinking water reservoir (Yuqiao) in Tianjin municipality, north-eastern China. The reservoir is eutrophic with annual algae blooms in late summer. The watershed (∼436 km2) has a sub-humid continental monsoon climate, with an annual mean temperature of 14 °C and an average annual precipitation of 653 mm. Nearly three-fifths of this precipitation falls in the summer period between July and September.15 The area is characterized by a varied topography: lowlands (with an average gradient of less than 2°) and plains (2–6°) account for 22% and 25% of study area, respectively, thus together constituting nearly half of the watershed. Hilly land with gradients of 6–15°, low mountain regions with gradients of 15–25° and mountain region (>25°) account for the remaining 23%, 19% and 11% of the watershed, respectively. On a macro-scale the terrain raises from the south, where the Yuqiao reservoir is situated, towards the north. The soils are predominantly a mix of gleysols in the lowland and lithosols in the mountain. The gleysols are developed in deltaic alluvial sediments, while the lithosols are formed through weathering of the underlying parent sedimentary bedrock, consisting of sandstone and limestone. The approximately 130
000 people that reside within the study area have agriculture as their main livelihood. The lowland and plains are either used for growing crops, rotating between summer maize and winter wheat, or for vegetable farming. The hilly and mountainous areas are predominantly used for growing either fruits (orchards) or timber (forest). Throughout the catchment there is extensive animal farming. The local environmental protection bureau16 reports that applying excessive amounts of inorganic P fertilizers is the general practice in this region. Moreover, dung from the extensive husbandry is used as an organic fertilizer, increasing the P loading by a factor of three, to a staggering yearly total P application of 5.2 ∼ 35.8 g P to each square meter of agricultural land.
Processes governing mobilization and transport of P in the local watershed have been assessed through preceding extensive synoptic physicochemical studies of the soil and regional monitoring of water chemistry within the SinoTropia project.1 This study builds on and is an extension of these studies.
2.2 Soil sampling and pre-treatment produces
The selection strategy for the 56 soil samples used in this study aimed for an even spatial distribution as well as capturing the four major land use types in the region (Fig. 1). All samples were taken from the 0–15 cm mineral top soil A horizon (A) which had the largest P pools and most abundant content of P species.17,18 Soil samples were composed from 10 sub-samples according to a radial scheme.19 Soil sampling was conducted before planting and fertilizer application. Sample pre-treatment was conducted according to ISO11464.20 The samples were gently crushed by a mortar and pestle to break up clods and pass through a 2 mm sieve. Samples for phosphatase assay were stored field moist at 4 °C, while the rest of the samples were air dried and stored dark at room temperature.
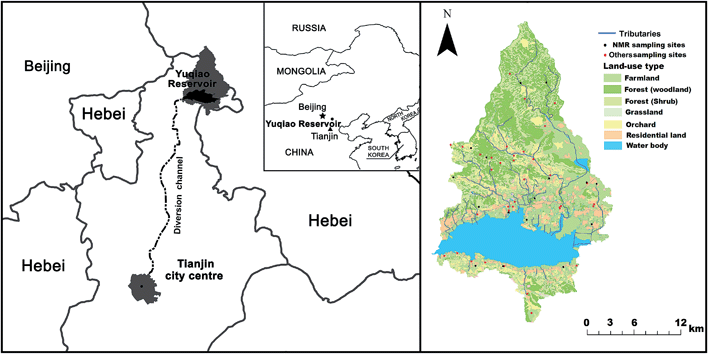 |
| Fig. 1 Location of the local watershed to the Yuqiao Reservoir, soil sampling sites (red dots) and sampling sites for NMR 31P analysis (black dots). | |
2.3 Soil analysis
2.3.1 General soil properties.
Soil pH in deionized water was determined following ISO10390.21 Organic matter content was measured gravimetrically according to Krogstad22 by determining the loss on ignition. Soil texture of the samples was determined by analyzing the particle size distribution (PSD) with a laser diffraction particle size analyzer (Beckman Coulter LS 13320). Sample preparation for the PSD analysis followed the procedure described by ISO11277.23 Effective cation exchange capacity (CECe) was determined following the method by Hendershot and Duquette,24 which is comparable to ISO11260.25 The exchanged acid- and base cations in the extracts were analyzed using Inductively Coupled Plasma Optic Emission Spectroscopy (ICP-OES).
2.3.2 Soil mineralogy.
The crystalline mineralogy of the soil was analyzed by an X-ray Diffraction (XRD) analyzer26 (Bruker D8). The mineral signals were interpreted by software TOPAS 4.2.27 Amorphous iron- and aluminum-oxyhydroxide were extracted with ammonium oxalate (Feox and Alox)28 and citrate–bicarbonate–dithionite (Fecbd, Alcbd),29 separately. The contents of Fe and Al in the extracts were analyzed using ICP-OES.
2.3.3 P pools and P-sorption indexes.
Extraction of organic and inorganic phosphorus pools in the soils was conducted according to the method described by Møberg and Petersen:30 total inorganic P (TIP) was extracted from the soils using hot (70 °C) 6 M sulfuric acid. The total P (TP) content was extracted in a similar manner after combustion of the soil sample at 550 °C. Total organic P (TOP) was calculated as the difference between TP and TIP. STP was extracted using the Mehlich-3 reagent.31 Phosphate concentration in all the extracts was determined spectroscopically using the molybdenum blue method according to ISO6878 (ref. 32) as described by G. M. Pierzynski.7
P sorption index (PSI), introduced by Bache and Williams,33 was developed further to rapidly estimate the additional soil P sorption capacity under the existing soil STP content level. It is determined as a single-point isotherm by mixing the soil with an excess of orthophosphate (1.5 mg P per g soil)33 and measuring the concentration of unsorbed P remaining in solution.34 The index is given as the ratio of sorbed (X in mg P kg−1) over the log concentration of unsorbed P (log
C in mg P L−1) according to eqn (1):
| 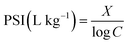 | (1) |
Bache and Williams33 suggested that the arbitrary numeric sum of PSI and STP gave a reasonable relative proxy for the total P sorption capacity (PSC) of the soil. The sum of soil PSI and STP is therefore applied as a reference for soil PSC in this study. The Degree of P Saturation (DPS) in the soils was thereby determined using eqn 2.2,35
| 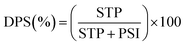 | (2) |
2.3.4 Soil P species.
Phosphorus species in the soils were determined using 31P-NMR. The species were extracted from the soils with a mixture of 0.25 M NaOH and 0.11 M EDTA,36,37 centrifuged at 10 000 g and a 5% (v/v) mixture of sodium carbonate and sodium dithionite was added prior to freeze drying.38 The freeze dried materials were transferred to a NMR tube and dissolved in NaOH and D2O. The spectra were obtained on a 400 Hz 31P-NMR spectrometer using 85% H3PO4 as an external standard (δ = 0 ppm) via the signal lock,39 and analyzed by MestReNova 8.1 software.40 Peak assignments for the spectra derived from the literature39,41–44 were orthophosphate (Ortho-P) at δ = 5.7–6.1 ppm, pyrophosphate (Pyro-P) at δ = −4 to −5 ppm, monoesters (monoester-P) at δ = 3 to 6 ppm, phosphonates at δ = 18 to 20 ppm, and terminal and middle polyphosphates (terminal Poly-P and middle Poly-P) at −4 ppm and −19 to −21 ppm, respectively. The total P contents in the NaOH and EDTA extracted NMR samples were determined using ICP-OES. The contents of different species are presented as their relative contribution to this total P.
2.3.5 Phosphatase activities.
The activities of four phosphatase enzymes were determined according to the method of Tabatabai.45 Both acid (AcP; EC3.1.3.2) and alkaline phosphomonoesterases (AlP; EC3.1.3.1) in field moist soil samples were incubated using p-nitrophenyl phosphate (PNP) as the substrate in their modified universal buffer with pH values of 6.5 and 11, respectively. Phosphodiesterase (PD; EC3.1.4.1) was assayed with bis-p-nitrophenyl phosphate (BPNP) as the substrate with a buffer pH of 8. AcP, AlP and PD activities were expressed as the content of p-nitrophenyl, which was measured spectroscopically at λ = 410 nm. Their activities are therefore expressed as mg p-nitrophenyl kg−1 soil (dry weight) h−1. Inorganic pyrophosphatase (PY; EC3.6.1.1) was assayed using sodium pyrophosphate decahydrate as the substrate. The assay of PY activity was based on the determination of the amount of orthophosphate released when soil is incubated with buffered (pH 8.0) pyrophosphate solution, which was measured spectroscopically at λ = 880 nm. PY activity was expressed as mg PO43−–P kg−1 h−1.
2.4 Statistical analysis
Cluster analysis was applied to identify similarities in variability among variables (soil properties, land use and soil P pools) using Minitab 16.0 software. The similarities are based on a measure of the Euclidean distance. A one-way ANOVA with Duncan test (p = 0.05) was applied to determine significant differences between group means using SPSS 17.0.
3 Results and discussion
3.1 Soil properties and P pools
Average physicochemical soil properties and sizes of soil P pools from the four different land use categories are given in Table 1. Soil pH values are neutral or slightly alkaline, with no significant differences between the land use categories. This circumneutral pH is consistent with previous research on soil pH in northern China46 and is due to buffering by carbonate minerals in the predominant sedimentary bedrock. An overall low soil organic matter content (SOM), in contrast, differs noticeably between the land use categories. This is likely due to the different agricultural management practices among the land use categories. SOM is relatively high in the forest area (6.8%) and lowest in the cropland (3.5%). SOM in the forest soils is mainly derived from the incomplete decomposition and humication of litterfall from the forest canopy. As for cropland, the long-term practice of crop harvesting with removal of the whole plant leads eventually to a depletion of SOM.47 A relatively high SOM content was found in the vegetable fields. This is mainly due to the copious application of organic fertilizers (sewage and manure) and plant residues from crop production in these soils.
Table 1 Soil physicochemical characteristics and P pools of each land use typea
Land-use |
Horizon |
pH (H2O) |
Organic matter (%) |
Soil texture (volume%) |
Slope (%) |
P pools mg P kg−1 |
Clay |
Silt |
Sand |
TIP |
TOP |
Values are the mean ± standard deviation, and values within a row for each property with the same letter are not significant at the 5% level (Duncan).
|
Forest |
A |
7.0 ± 1.2a |
6.8 ± 2.2c |
1.8 ± 0.7a |
56 ± 1.5a |
42 ± 1.5c |
11.1 ± 0.8b |
248 ± 13a |
167 ± 7a |
Orchard |
A |
7.3 ± 0.8a |
4.2 ± 1.5ab |
1.7 ± 0.8a |
61 ± 1ab |
37 ± 1.1bc |
7 ± 1.1ab |
537 ± 35ab |
229 ± 16ab |
Cropland |
Ap |
7.2 ± 0.8a |
3.5 ± 0.4a |
2.5 ± 0.4b |
70.9 ± 0.6c |
26.4 ± 0.6a |
4.7 ± 0.4a |
638 ± 20b |
203 ± 15ab |
Vegetable fields |
Ap |
7.2 ± 1a |
5.2 ± 3.2b |
2.7 ± 0.6b |
67.9 ± 0.7bc |
29.6 ± 0.7ab |
4.8 ± 0.2a |
993 ± 70c |
543 ± 38b |
The soils had a relatively homogeneous particular size distribution (PSD) with silty loam as the main soil texture category. This texture indicates a relatively easily eroded soil with poor P adsorption capacity.48,49 Compared to forest and orchard soils, the cropland and vegetable field soils hold a somewhat higher content of fine material (clay and silt). This is most likely the cause for the selected land use by the farmer, rather than an effect. Moreover, cropland and vegetable fields are mainly established on the fluvial flood plains, which are inherently richer in fine material, while orchards and forests are planted on soils developed on steep slopes, from which fine material is efficiently flushed. Total inorganic P (TIP) constitutes consistently the largest P pool, which differs substantially between the different land use categories. This is clearly attributed to the agricultural practices, pertaining especially to the general practice of applying excessive amounts of inorganic P fertilizers to the cropland and vegetation fields. In general, the soil content of TIP increases in the following order: forest < orchard < cropland < vegetable fields. This is consistent with local management data on agricultural practices regarding the applied amounts of P fertilizers. The overall content of total organic P (TOP) was significantly less than TIP in all land use categories. Forest soil showed the lowest TOP content (167 mg P kg−1) due to the minor or no application of P fertilizers. TOP did not differ significantly between the orchard and cropland, though the levels in cropland were slightly lower, despite higher loading of P fertilizers. This is likely due to the lower SOM content, as discussed above, which inherently will contribute to less TOP content in the cropland soils. Vegetable fields showed a relatively high level of TOP (543 mg P kg−1), reflecting both the overall high loading of P fertilizers and the slightly higher SOM due to the crop residues and relatively larger amounts of organic fertilizers applied to these soils.
3.2 Effective cation exchange capacity (CECe) and composition
Generally, CECe and its composition did not differ significantly between the four land use categories. The base saturation in the soils is high (>85%) with calcium and magnesium being the main exchangeable cations, accounting for 90% of the CECe. The acid cations aluminum and manganese thus contributed much less to the cation exchange capacity (less than 7 cmol kg−1). Moreover, exchangeable iron was not detected in any of the soils. This implies that the prevalent carbonate weathering process is rather uniform within the study area and that the influence of agricultural activities on the soil CECe composition is limited. Moreover, the high content of exchangeable divalent base cations indicates that the PO4 solubility in soil solution is controlled by saturation with calcium and magnesium compounds, such as di- and tricalcium phosphate, through the precipitation reaction.
3.3 Soil mineral composition
The crystalline mineral compositions were found to be rather homogeneously distributed in the soils. Quartz (33–39%), halloysite (21–24%) and muscovite (20–27%) were the main components. In addition, some albite (4–7%) was identified in most of the soil samples. Phosphorus containing minerals, such as apatite and vivianite, were not found in any of the soil samples, despite the omnipresence of sedimentary deposits and sedimentary minerals. This was also reported by a local soil nutrient survey.50 This implies that the P in the soil is mainly from agricultural practices.
3.4 Iron and aluminum oxides
The contents of two different pools of Fe- and Al oxides in the soil are presented in Table 2. Amorphous iron (Feox) and aluminium (Alox) are present in similar amounts within each land use category. Their average contents were slightly higher in the cropland and vegetable field relative to the forest and orchard. This is likely mainly related to differences in soil-forming factors, especially time and weathering conditions51 between the lowlands and mountainous regions. Some studies52–54 have found that the content of amorphous Fe and Al oxides may also be influenced by long-term agricultural practices by changing the SOM level, which might have an inhibiting effect on the crystallization.53,54.
Table 2 Iron and aluminium extractable in ammonium oxalate (Alox, Feox) and citrate-bicarbonate-dithionite (Fecbd, Alcbd)a
Land-use |
Horizon |
Alox |
Feox |
Alcbd |
Fecbd |
(mmol kg−1 soil) |
Values are mean ± standard deviation, and values within a row for each property with the same letter are not significant at the 5% level (Duncan).
|
Forest (n = 14) |
A |
52 ± 8a |
50 ± 10a |
54 ± 8b |
208 ± 22b |
Orchard (n = 14) |
A |
48 ± 6a |
48 ± 12a |
41 ± 5ab |
215 ± 25b |
Cropland (n = 14) |
Ap |
55 ± 6b |
56 ± 5b |
36 ± 3a |
183 ± 10a |
Vegetable fields (n = 14) |
Ap |
58 ± 6b |
58 ± 8b |
34 ± 3a |
182 ± 18a |
Amorphous Fe and Al oxide55 forms have greater P sorption capacity than their well-crystalline forms due to their higher specific surface area.56,57 The Feox and Alox are therefore likely explanatory factors for the differences in P sorption capacity between the land use categories (Fig. 2c). The CBD extractable iron (Fecbd) values are generally higher than Feox oxides indicating the dominance of crystalline Fe over amorphous forms. This is also found elsewhere in other studies.58,59 Alcbd was not present at a higher level than Alox. The ratio between average Alox and Alcbd ranged from 0.96 to 1.7. These high ratios reflect that Al is mainly present in amorphous forms.60,61
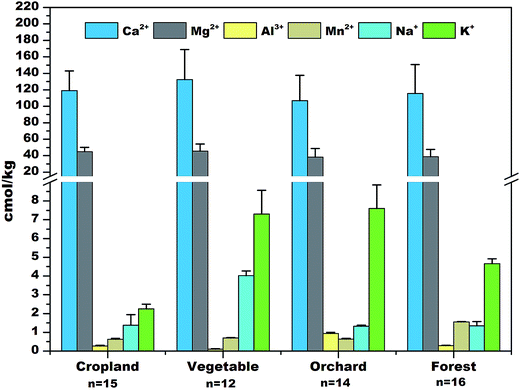 |
| Fig. 2 CECe in soils from four land use categories (same letter denotes no significant difference at the 5% significance level (Duncan) and error bars around means present the standard deviations). | |
3.5 P sorption indices
The amount of STP measured in cropland and vegetable field soils was significantly higher than in the orchard and forest soils (Fig. 3). The mean value of the STP content in the forest soil was less than 20 mg kg−1, while it ranged from 90 mg kg−1 to 410 mg kg−1 in the soil from the vegetable fields. This was as expected considering that background values of natural P are low and that the STP levels in the cropland and vegetable fields are significantly affected by the long term and recent application of excess P fertilizers.
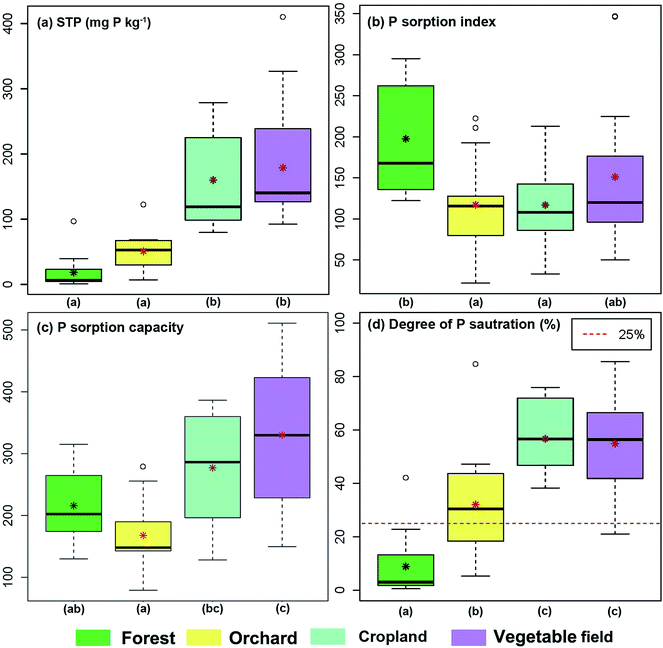 |
| Fig. 3 Box plot of potential phosphorus loss indices: soil test P (STP), P sorption index (PSI), P sorption capacity (PSC) and degree of P saturation (DPS). Red stars denote the mean value. Land use with the same letter within each parameter is not significant at the 5% level (Duncan). Open circles denote outliers. | |
The low PSI values (Fig. 3b) imply that the soils have very poor P sorption capacities. The cause for this is partly owing to few adsorption sites, due to the low SOM content, coarse soil texture and predominance of 1
:
1 type clays, and partly due to the heavy loading of P causing a high PO4 concentration in the soil solution. The PSI values of forest soils were higher than in the other land use types. This could be partly due to that only a limited number of the available P sorption sites were occupied, and partly due to the higher SOM content, increasing the sorption capacity of the soil through cation bridging mechanism. Phosphate binds to SOM through ternary complexes, with cations like Fe(III) and Al(III) serving as bridging metal centers between phosphate anions and the negatively charged organic functional groups.62–66 Orchard, cropland and vegetable field soils possessed rather similar PSI values. The long term agricultural management practice in these fields has likely slightly altered the soil properties of these soils, especially for the relatively coarse-textured soils.67 This is likely the cause for the slightly higher PSI values in the agricultural soils compared to the soils in the croplands.
PSC values (Fig. 3c) in the cropland and vegetable fields were higher than those in the forest and orchard. This may partly be due to the relatively higher content of amorphous Al and Fe oxides in the cropland and vegetable field (Table 2), increasing the P sorption capacity.56,57 It may also partly be owing to slightly higher clay contents, which is commonly related to higher specific surface areas, although 1
:
1 type clays predominate.
DPS is a proxy for the degree of soil P saturation and is used to predict risks for P loss.68 Previous studies have suggested a DPS of 25% as a critical threshold value, above which the potential for P loss becomes unacceptable.69,70 The mean DPS values for cropland and vegetable field soils in the study site were 55% and 57%, respectively, while the forest soils had DPS values that were consistently (except one outlier) below 25% (Fig. 3d). These DPS results thus document that there is an unacceptable potential for P loss from all the studied land use categories with the exception of forests. In general, the differences in DPS among the land uses are consistent with the locally recorded amount of applied fertilizers. It is noteworthy that the croplands and vegetable fields, consisting of soils with high potential for P loss, are mainly located in the plain area adjacent to the surface water reservoir suffering eutrophication.71
3.6 Statistical analysis
A dendrogram of the explanatory parameters (land use types, organic matter content, soil texture, amorphous Fe and Al oxides and slope) along with the response parameters (P pools, STP, PSI and PSC) is shown in Fig. 4. All parameters are grouped into two main clusters, each with two sub-groups, based on respective similarities. Moreover, the four land use types are assigned to each of the four sub-groups. Cluster 1, containing the groupings with orchard and forest soils, showed high similarities with the explanatory parameters pH, sand, silt, SOM and slope, and the response parameter PSI. This is conceptually sound as the forests and orchards are mainly located on steep slopes and on poor soils having a relatively coarse soil texture attributed to the hydrological processes flushing out the finer particles. The only explanatory factor with a causal relationship to PSI is the SOM, which is relatively higher in forest and orchard soils. In addition, no or limited application of P fertilizers and manure to these fields is prone to have left more sorption sites available for P binding. In Cluster 2 the group of cropland and vegetable field soils showed stronger similarity with P sorption parameters and P pools, respectively. This testifies to the inherent relationship between intensive agricultural activities, causing large P pools and thus higher risk for P loss. Amorphous Al (Alox) and Fe (Feox) also showed a higher similarity within the vegetable and cropland group, which further accounts for the different PSC between land use categories (Fig. 3c). The figure illustrates that land use is an important explanatory proxy for the P response parameters. Based on this it is evident that land use data, with its intrinsic management practices, are the main collective explanatory factor to consider when assessing spatial distribution of risk for P loss.
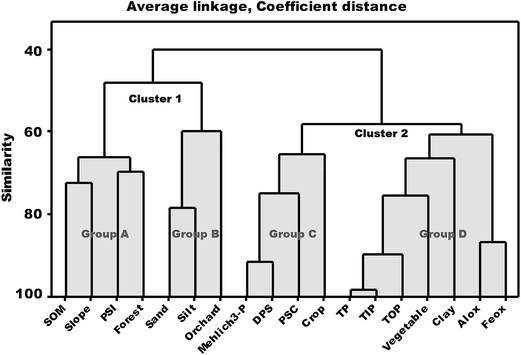 |
| Fig. 4 Dendrogram of all explanatory and response variables. | |
A Pearson correlation analysis was conducted in order to deduce empiric relationships between Fe and Al oxide forms and PSC. Both amorphous (Alox) and crystalline (Alcbd) aluminum oxide pools were found to be strongly correlated with PSC, with correlation coefficients (r) 0.86** and 0.69**, respectively. Rather surprisingly, amorphous iron (Feox) was found to be only moderately correlated with PSC (r = 0.51**) and the pool of crystalline iron (Fecbd) was not significantly correlated with PSC. This indicates that Al oxides may play a more important role in P sorption than Fe oxides in this study. This is also found in other studies.72–74 On the other hand, the sum of Feox and Alox presents significant correlation with PSC (r = 0.70**). Most of the variation in the P sorption capacity is thus empirically explained by the total amount of amorphous Fe and Al oxides, which is in agreement with the conceptual understanding.
3.7 Phosphorus species
The concentration and distribution of P species in each of the land use categories are given in Table 2, and four representative 31P NMR spectra for each land use type are shown in Fig. 5. Orthophosphate is the predominant P species, accounting for 62–93% of the total P extracted (NE-TP) from the soils. This is consistent with findings in other studies.75,76 There is a clear gradient in the absolute and relative contents of orthophosphate between the four land use categories. Generally the orthophosphate contents and their ratios to NE-TP increased with increasing agricultural management intensity: the mean orthophosphate level in forest soil was only 97 mg P kg−1, while the values for cropland and vegetable field soils were 333 and 771 mg P kg−1, respectively (Table 3).
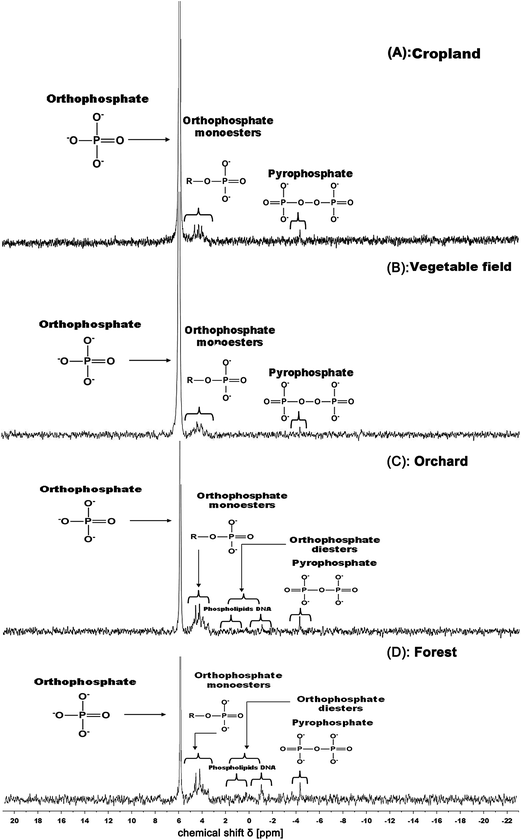 |
| Fig. 5 Selected 31P NMR spectra of NaOH–EDTA extracts of the studied four land-use types. | |
Table 3 Concentrations (mg P kg−1 soil) of different P species, measured using 31P NMR, within different land-use types and their proportions to total P in NaOH and EDTA extractsa
Land-use |
NE-TP |
NMR-Pi |
NMR-Po |
Ortho-P |
Pyro-P |
Poly-P |
Monoester-P |
Diester-P |
Phos-P |
Total monoester-P |
Inositol-P |
PL |
DNA-P |
Myo- |
Scyllo- |
NE-TP, total P in NaOH and EDTA extracts; NMR-Pi, inorganic P in NaOH and EDTA extracts; NMR-Po, organic P in NaOH and EDTA extracts; Ortho-P, orthophosphate; Pyro-P, pyrophosphate; Poly-P, polyphosphates; monoester-P, orthophosphate monoesters (orthophosphate monoesters include inositol-P); inositol-P, inositol phosphate; Myo-, myo-inositol; Scyllo-, scyllo-inositol; Diester-P, orthophosphate diesters; PL, phospholipid; Phos-P, phosphonates; i, ii and iii represent the number of detected samples (1, 2 and 3); parentheses indicate the average proportion to total P in NaOH and EDTA extracts; the values within a row for each property with the same letter are not significant at the 5% level (Duncan).
|
Forest |
155 ± 12a |
79 ± 7a, (50.7%) |
7 ± 0.6b, (4.5%) |
2.3 ± 0.2ab, (1.5%) |
63 ± 5a, (40.9%) |
4.4 ± 0.4b, (2.8%) |
2.4ii, (0.8%) |
1.3 ± 0.5iii, (0.8%) |
0.7 ± 0.01, (0.5%) |
2.1 ± 0.2, (1.4%) |
Orchard |
374 ± 15b |
287 ± 14b, (76.7%) |
3 ± 0.5a, (0.8%) |
1.5 ± 0.1a, (0.4%) |
80 ± 6c, (21.5%) |
3.1 ± 0.2ab, (0.8%) |
3.0ii, (0.8%) |
1.6 ± 0.4, (0.4%) |
0.1 ± 0.02, (0.03%) |
1.1ii, (0.3%) |
Cropland |
409 ± 127c |
360 ± 13b, (88.1%) |
2.9 ± 0.1a, (0.7%) |
2.3 ± 0.3ab, (0.6%) |
39 ± 6bc, (9.7%) |
5.8 ± 0.3c, (1.4%) |
2.8i, (1.2%) |
2.5 ± 0.3, (0.9%) |
0.4 ± 0.03iii, (0.2%) |
1.4ii, (0.3%) |
Vegetable fields |
826 ± 47d |
770 ± 43c, (93.3%) |
2.9 ± 0.2a, (0.4%) |
1.3 ± 0.1a, (0.2%) |
49 ± 3b, (5.9%) |
2.5 ± 0.2a, (0.3%) |
0.9i, (0.1%) |
1 ± 0.4, (0.1%) |
0.2 ± 0.01, (0.02%) |
1.2 ± 0.05iii, (0.1%) |
There were no clear differences in the overall low (around 3 mg P kg−1) pyrophosphate content in the orchard, cropland and vegetable field soils. By contrast, the average pyrophosphate content of forest soils was relatively high, i.e. 7 mg P kg−1, accounting for 4.5% of NE-TP. This is most likely due to differences in the content of soil microorganisms, as pyrophosphate in soils is generally considered to be the product of their metabolism.77 In general, undisturbed soils commonly contain more soil microorganisms since the amount, as well as species diversity, of microorganisms is reduced by agricultural management.78
The average content of polyphosphate was less than 2.3 mg P kg−1. This extremely low level of polyphosphate in the soils may be due to its poor stability (half-life = 0.8 year).41 Under alkaline conditions, polyphosphate may be completely hydrolyzed into pyrophosphate and even partially to orthophosphate.76,79 The addition of NaOH–EDTA, used as an extraction solution, may thus result in de-polymerization of polyphosphate.
Orthophosphate monoester-P was the major soil organic P (OP) species in the soils, accounting for between 6 and 29% of NE-TP. The content of monoester-P in the soil was found to decrease with increasing intensity of agricultural management (i.e. forest > orchard > cropland > vegetable fields). This negative relationship is also found in other studies80,81 and is explained by increased soil disturbance with increasing management intensity.
Generally, inositol phosphates are considered to be rather non-bioavailable due to their strong binding to clay, organic matter and metal oxides in the soils.82,83 On the other hand, inositol phosphates are gaining increased attention due to their potential bioavailability once in solution and subsequent contribution to eutrophication.84 The general content of inositol phosphates was found to be relatively low, with average values ranging from 0.9 to 5.8 mg P kg−1 (Table 2). This is especially the case for scyllo-inositol phosphates, which were only detected in few samples. The low content of inositol phosphates may relate to the relatively low vegetation abundance or the poor adsorption capacity of the soils due to the generally low clay and organic matter content.84 On the other hand, considering that monoester phosphates are highly labile, it is conceivable that they constitute the majority of the monoester phosphate group in the fresh soil sample, even though they were not detected in the stored and pre-treated sample material.
The orthophosphate diester group, being the backbone of DNA and central in phospholipids, also showed a relatively low content in the soils. Turner and Engelbrecht85 reported that DNA is liable to accumulate in cold, wet and acidic soils, while relatively high soil pH conditions enhance the degradation of DNA. It is worth mentioning that RNA was not detected in practically any of the soils. This is most likely due to the rapid hydrolysis of RNA under the alkaline conditions generated by NaOH–EDTA extraction.43
Similar to the orthophosphate diester, phosphonates were also only found at relatively low concentrations. This could again be due to their poor stability in alkaline solution as well as their inefficient extraction.41
The consistent trend of absolute and relative increase in orthophosphate and decrease in monoester-P with increasing intensity of agricultural management, among the four land use categories, substantiates land use as a key explanatory factor for the spatial variation in soil phosphate chemistry.
3.8 Soil phosphatase activities
Phosphatase activities have been granted increased consideration within the fields of agriculture and environment in recent years.86 This is mainly due to their catalysis of the hydrolysis of ester–phosphate bonds, leading to the release of orthophosphate.87 The activity of this enzyme needs therefore to be considered as an explanatory parameter when assessing the hydrolysis of soil organic P, and thereby its environmental impact.88 Phosphatase activities were found to be generally higher in forest and orchard soils than in cropland and vegetation fields (Fig. 6). Tarafdar and Claassen89 reported that phosphatases found in the soil is mainly from microbial organisms. As argued above, the uncultivated or less cultivated soils commonly have higher microbial activities due to less human distortions. Relatively low levels of acid phosphomonoesterases (AcP) and high levels of alkaline phosphomonoesterases (AlP) were found. The governing factor here is most likely the circumneutral soil pH. Barbarić, et al.90 reported that acid phosphatases have optimal activity at pH values from 3 to 4.5, while the average pH value of these soils was around 7, which is more suitable for the production of AlP.
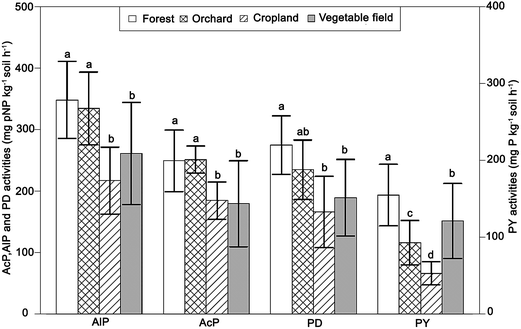 |
| Fig. 6 Soil phosphatase activities in soils from four land use categories (AlP, alkaline phosphomonoesterases; AcP, acid phosphomonoesterases; PD, phosphodiesterases; pyrophosphatase; same letter denotes no significant difference at the 5% significance level (Duncan) and error bars around means present the standard deviations). | |
Phosphodiesterase (PD) and pyrophosphatase (PY) activities were generally lower than that of AlP. The cause for this is most likely related to the limited availability of the respective substrates in the soils (Table 2).
There is no clear consistent trend in phosphatase activities between the four different land use types, though forest and orchard soils showed similar and relatively high phosphatase activities, whereas cropland and vegetable field soils had relatively low activities. The results indicate therefore that phosphatase activities were generally higher in uncultivated soils than in cultivated soils, which is consistent with previous studies.6,91
3.9 Effects of agricultural practices on the potential risk for P loss
This study confirms that the difference in agricultural management practices is the main cause for the spatial variation in soil P pools. It is clear that the cumulative effect of excess application of P fertilizers has inherently increased the total P, inorganic P fractions and STP in the soils, in accordance with the following order of land use: vegetable fields > cropland > orchard > forest soils. Furthermore, DPS, an indicator for assessing the risk for loss of soil P, constitutes a large environmental risk as its critical threshold value of 25% is greatly exceeded, except for forest soils. The DPS is strongly correlated with the amount of STP between and within the four land use types. This indicates that the application of P, as the dominating source of STP, is the direct cause for the increased potential risk for loss of P. Moreover, from the perspective of spatial distribution, most cropland and vegetable fields are located in the vicinity of rivers or surface waters. These regions should be given special attention considering the potential environmental risk of these hot-spots for P loss.
Orthophosphate is the dominant P species in all studied soils, constituting from 62% to 93% of the extracted total P. The levels of orthophosphate were especially high in soils from croplands and vegetable fields subject to intensive application of P fertilizers and manure. Orthophosphate monoesters, which are the dominating organic P moieties, revealed a declining trend with increasing agricultural management practices. Higher phosphatase activities were thus generally found in forest and orchard soils. This was especially the case for AlP activities which averaged around 340 mg pNP kg−1 h−1 in forest and orchard soils. In soils with high phosphatase activity the orthophosphate monoesters are catalytically hydrolyzed to free phosphates, constituting an important source of bioavailable P.14 Orchard soils should therefore be given increased attention considering their relatively high content of orthophosphate monoesters and AlP activities.
4. Conclusions
Our results confirm that the soil P pools and P species are significantly affected by long-term application of excess mineral- and organic fertilizers. TP, TIP and STP showed a consistent increasing trend between different land use categories, in the following order: forest < orchard < cropland < vegetable field soils. This land use sequence is consistent with the degree of agricultural management intensity including application of P fertilizers.
Relatively coarse soil texture, predominance of 1
:
1 type clays and poor organic matter content render the soils with poor P sorption capacity. This together with a heavy loading of P caused STP to hold strong explanatory power as a DPS indicator. Considering the measured DPS values relative to its critical threshold value (25%) reveals a large potential risk for soil P loss from cropland and vegetable field soils. Moreover, these intensively cultivated lands are normally situated in close proximity to the surface water reservoirs.
Orthophosphate and monoester-P were found to be the major P species. Long-term intensive agricultural practice of excess P application is believed to have caused the large orthophosphate pools and the low content of monoester-P in the soils. Moreover, soil phosphatase activities were higher in forest and orchard soils than in cropland and vegetable field. AlP constituted the strongest phosphatase activity due to a circumneutral soil pH. This implies that it may constitute a potential environmental risk through the hydrolysis of monoester-P. Orchard soils possess both high monoester-P content and AlP. Based on this it is recommended to the authorities to pay more attention to the orchard field in our study area. These results demonstrate that together with regionalized studies, the type of land use can be generally used as the explanatory factor for assessing potential risks of phosphorus loss from soils.
Acknowledgements
The authors are grateful to the Research Council of Norway for funding the SinoTropia Project (Project no. 209687/E40). The authors would also like to acknowledge the numerous local farmers for their kind assistance during the fieldwork.
References
- D. L. Correll, J. Environ. Qual., 1998, 27, 261–266 CrossRef CAS.
- Y. Wang, The University of Guelph, 2010.
- L. J. Agnew, S. Lyon, P. Gérard-Marchant, V. B. Collins, A. J. Lembo, T. S. Steenhuis and M. T. Walter, J. Environ. Manage., 2006, 78, 63–76 CrossRef PubMed.
- H. Elliott, R. Brandt, P. Kleinman, A. Sharpley and D. Beegle, J. Environ. Qual., 2006, 35, 2195–2201 CrossRef CAS PubMed.
- A. N. Sharpley, R. W. McDowell and P. J. Kleinman, Plant Soil, 2001, 237, 287–307 CrossRef CAS.
- A. Zhang, Z. Chen, G. Zhang, L. Chen and Z. Wu, Eur. J. Soil Biol., 2012, 52, 73–77 CrossRef CAS PubMed.
- G. M. Pierzynski, North Carolina State University Raleigh, 2000.
- P. D. Schroeder, D. E. Radcliffe, M. L. Cabrera and C. D. Belew, J. Environ. Qual., 2004, 33, 1452–1463 CrossRef CAS.
- A. N. Sharpley, J. Environ. Qual., 1995, 24, 920–926 CrossRef CAS.
- Y. Wu, J. Prietzel, J. Zhou, H. Bing, J. Luo, D. Yu, S. Sun, J. Liang and H. Sun, Sci. China: Earth Sci., 2014, 1–9 Search PubMed.
-
B. J. Cade-Menun, Organic phosphorus in the environment, 2005, pp. 21–44 Search PubMed.
- O. Borggaard, cta Agric. Scand., 1986, 36, 107–118 CrossRef CAS PubMed.
- O. Borggaard, J. Soil Sci., 1983, 34, 333–341 CrossRef CAS PubMed.
-
B. L. Turner, E. Frossard and D. S. Baldwin, Organic phosphorus in the environment, CABI, 2005 Search PubMed.
-
JCBS, Ji County Statistical Yearbook 2011, Ji county bureau of statistics, 2011 Search PubMed.
-
JCEPB, Ji County Environmental Quality Report (2012), Ji county environmental protection bureau, 2012 Search PubMed.
- K. Kitayama, N. Majalap-Lee and S.-i. Aiba, Oecologia, 2000, 123, 342–349 CrossRef.
-
E. Pettersen, MsC, University of Oslo, 2014.
- L. Wilding, 1985.
- ISO11464, International Organization of Standarization, Switzerland, 2006.
- ISO10390, International Organization of Standardization, Switzerland, 2005.
-
T. Krogstad, NLH report no. 6 institutt for jordfag, Norsk Landbrukshøyskole, 1992, p. 32s Search PubMed.
- ISO11277, International Organization of Standardization, Switzerland, 2009.
- W. H. Hendershot and M. Duquette, Soil Sci. Soc. Am. J., 1986, 50, 605–608 CrossRef.
-
I. 11260, Soil Quality: Determination of Effective Cation Exchange Capacity and Base Saturation Level Using Barium Chloride Solution, ISO, 1994 Search PubMed.
-
W. Harris and G. N. White, Methods of soil analysis: Part, 2008, vol. 5, pp. 81–115 Search PubMed.
- D. T. BrukerAXS, Bruker-AXS GmbH, Karlsruhe, Germany, 2008.
- J. McKeague and J. H. Day, Can. J. Soil Sci., 1966, 46, 13–22 CrossRef CAS PubMed.
- O. Mehra and M. Jackson, 1958.
-
J. P. Møberg and L. Petersen, Øvelsesvejledning til geologi og jordbundslære 2, Den kgl. Veterinær-og Landbohøjskole, Kemisk Institut, 1982 Search PubMed.
- A. Mehlich, Commun. Soil Sci. Plant Anal., 1984, 15, 1409–1416 CrossRef CAS PubMed.
- ISO6878, International Organization of Standardization, Switzerland, 2004.
- B. Bache and E. Williams, J. Soil Sci., 1971, 22, 289–301 CrossRef CAS PubMed.
-
J. T. Sims, Methods of phosphorus analysis for soils, sediments, residuals, and waters, 2000, p. 22 Search PubMed.
- Y. Wang, T. Zhang, Q. Hu, C. Tan, I. Halloran, C. Drury, D. Reid, B. L. Ma, B. Ball-Coelho and J. Lauzon, J. Environ. Qual., 2010, 39, 1771–1781 CrossRef CAS.
- B. L. Turner, Eur. J. Soil Sci., 2008, 59, 453–466 CrossRef CAS PubMed.
- B. Cade-Menun and C. Preston, Soil Sci., 1996, 161, 770–785 CrossRef CAS PubMed.
- B. L. Turner, B. J. Cade-Menun and D. T. Westermann, Soil Sci. Soc. Am. J., 2003, 67, 1168–1179 CrossRef CAS.
- B. J. Cade-Menun, Talanta, 2005, 66, 359–371 CrossRef CAS PubMed.
- M. R. Willcott, J. Am. Chem. Soc., 2009, 131, 13180 CrossRef CAS.
- L. Zhang, S. Wang, L. Jiao, Z. Ni, H. Xi, J. Liao and C. Zhu, Ecol. Eng., 2013, 60, 336–343 CrossRef PubMed.
- W. Zhang, H. Zhang, W. Tang and B. Shan, Biogeosciences Discussions, 2013, 10, 16269–16294 CrossRef.
- B. L. Turner, N. Mahieu and L. M. Condron, Soil Sci. Soc. Am. J., 2003, 67, 497–510 CrossRef CAS.
- B. L. Turner and A. E. Richardson, Soil Sci. Soc. Am. J., 2004, 68, 802–808 CrossRef CAS.
-
M. A. Tabatabai, in Methods of Soil Analysis, Part 2-Micorbiological and Biochemical Properties, ed. A. R. W. Weaver and J. S. Bottomley, SSSA Book Series 1994, pp. 775–833 Search PubMed.
-
Y. Hseung, Report, 1980, p. 11 Search PubMed.
- D. Wright, J. Marois, M. Vargas and P. Wiatrak, 2003.
-
Y. Le Bissonnais, Soil erosion, conservation, and rehabilitation, 1996, pp. 41–60 Search PubMed.
- J. Sims, R. Simard and B. Joern, J. Environ. Qual., 1998, 27, 277–293 CrossRef CAS.
-
J. Wang, Ji County Soil Census Report, Ji County agricultural committee, 1982 Search PubMed.
- B. A. Stewart, Soil Sci., 1988, 145, 233 CrossRef PubMed.
- O. Borggaard, S. Jdrgensen, J. Moberg and B. Raben-Lange, J. Soil Sci., 1990, 41, 443–449 CrossRef CAS PubMed.
- V. U. Schwertmann, Z. Pflanzenernaehr., Dueng., Bodenkd., 1964, 105, 194–202 CrossRef PubMed.
- D. Nayak, D. Sarkar, K. Das and S. Chatterjee, J. Indian Soc. Soil Sci., 1999, 47, 322–328 Search PubMed.
- R. Cornell and U. Schwertmann, VCH Verlagsgesellshaft GMBH Weinheim, Germany, 1996.
- N. Nilsson, L. Lövgren and S. Sjöberg, Chem. Speciation Bioavailability, 1992, 4, 121–130 CAS.
- J. Torrent, V. Barron and U. Schwertmann, Soil Sci. Soc. Am. J., 1990, 54, 1007–1012 CrossRef.
-
T. Kparmwang, PhD. Thesis Ahmadu Bello University Zaria, 1993.
- B. Raji, I. Esu, V. Chude and J. Samara, Agrie. Res., 2000, 16, 41–51 Search PubMed.
- R. Parfitt and C. Childs, Soil Res., 1988, 26, 121–144 CrossRef CAS.
- K. Börling, E. Otabbong and E. Barberis, Nutr. Cycling Agroecosyst., 2001, 59, 39–46 CrossRef.
- R. Haynes and R. Swift, J. Soil Sci., 1989, 40, 773–781 CrossRef CAS PubMed.
- J. Gerke and R. Hermann, Z. Pflanzenernaehr. Bodenkd., 1992, 155, 233–236 CrossRef CAS PubMed.
- P. Bloom, Soil Sci. Soc. Am. J., 1981, 45, 267–272 CrossRef CAS.
- J. Gerke, Geoderma, 1993, 59, 279–288 CrossRef CAS.
- J. Gerke, Z. Pflanzenernaehr. Bodenkd., 1993, 156, 253–257 CrossRef CAS PubMed.
- C. J. Rosen and P. M. Bierman, University of Minnesota, Extension
Service. USA, 2005.
- M. RennesonA, 2010.
-
A. Breeuwsma, J. Reijerink and O. Schoumans, Animal waste and the land-water interface, S, 1995, pp. 239–249 Search PubMed.
- O. Schoumans and P. Groenendijk, J. Environ. Qual., 2000, 29, 111–116 CrossRef CAS.
- B. Zhou, R. D. Vogt, C. Xu, X. Lu, H. Xu, J. P. Bishnu and L. Zhu, Water, Air, Soil Pollut., 2014, 225, 16 Search PubMed.
- E. Williams, N. Scott and M. J. McDonald, J. Sci. Food Agric., 1958, 9, 551–559 CrossRef CAS PubMed.
- A. Kaila, 1963.
- H. Hartikainen, J. Sci. Agric. Soc. Finl., 1979, 51, 537–624 CAS.
- B. L. Turner, N. Mahieu and L. M. Condron, Org. Geochem., 2003, 34, 1199–1210 CrossRef CAS.
- K. Reitzel, J. Ahlgren, A. Gogoll and E. Rydin, Water Res., 2006, 40, 647–654 CrossRef CAS PubMed.
- C. Ghonsikar and R. Miller, Plant Soil, 1973, 38, 651–655 CrossRef CAS.
- Ö. Kara and İ. Bolat, Turk. J. Agric. For., 2008, 32, 281–288 Search PubMed.
- J. Ahlgren, L. Tranvik, A. Gogoll, M. Waldebäck, K. Markides and E. Rydin, Environ. Sci. Technol., 2005, 39, 867–872 CrossRef CAS.
- L. Condron, E. Frossard, H. Tiessen, R. Newmans and J. Stewart, J. Soil Sci., 1990, 41, 41–50 CrossRef CAS PubMed.
- R. McDowell and I. Stewart, Geoderma, 2006, 130, 176–189 CrossRef CAS PubMed.
- A. B. Leytem and R. O. Maguire, Inositol Phosphates, 2007, 150 Search PubMed.
- B. L. Turner, N. Mahieu, L. M. Condron and C. Chen, Soil Biol. Biochem., 2005, 37, 2155–2158 CrossRef CAS PubMed.
- B. L. Turner, M. J. Paphazy, P. M. Haygarth and I. D. McKelvie, Phil. Trans. Roy. Soc. Lond. B Biol. Sci., 2002, 357, 449–469 CrossRef CAS PubMed.
- B. L. Turner and B. M. Engelbrecht, Biogeochemistry, 2011, 103, 297–315 CrossRef CAS.
- M. Tabatabai and M. Fu, Soil Biochem., 1992, 7, 197–227 CAS.
-
P. Nannipieri, L. Giagnoni, L. Landi and G. Renella, in Phosphorus in action, Springer, 2011, pp. 215–243 Search PubMed.
- S. Newman, P. McCormick and J. Backus, J. Appl. Phycol., 2003, 15, 45–59 CrossRef CAS.
- J. Tarafdar and N. Claassen, Biol. Fertil. Soils, 1988, 5, 308–312 CrossRef CAS.
- S. Barbarić, B. Kozulić, B. Ries and P. Mildner, J. Biol. Chem., 1984, 259, 878–883 Search PubMed.
- V. Acosta-Martinez, L. Cruz, D. Sotomayor-Ramirez and L. Pérez-Alegría, Appl. Soil Ecol., 2007, 35, 35–45 CrossRef PubMed.
|
This journal is © The Royal Society of Chemistry 2015 |
Click here to see how this site uses Cookies. View our privacy policy here.