Dehydration of cellulose to levoglucosenone using polar aprotic solvents
Received
2nd February 2015
, Accepted 18th May 2015
First published on 18th May 2015
Abstract
Herein, we report an approach to produce levoglucosenone (LGO) from cellulose in yields up to 51% under mild reaction conditions (170–230 °C; 5–20 mM H2SO4) using polar, aprotic solvents such as tetrahydrofuran (THF). LGO can be used to make a wide variety of chemicals from biomass. The water content and solvent used in the reaction system control the product distribution. LGO is produced from the dehydration of levoglucosan (LGA). LGA is produced from cellulose depolymerization. Increasing the water content leads to the production of 5-hydroxymethyl furfural (HMF), obtaining a maximum HMF yield of 30%.
Broader context
This article describes the sustainable conversion of cellulose to levoglucosenone (LGO) using mild reaction conditions in the liquid phase. LGO is an attractive, biomass-derived platform molecule that can be used for the renewable production of pharmaceuticals, commodity chemicals such as 1,6-hexanediol (HDO), and green solvents such as dihydrolevoglucosenone. The co-production of such high-value species has the potential to significantly improve the overall economic feasibility of a biorefinery. Notably, dihydrolevoglucosenone, also known as Cyrene®, is a polar, aprotic solvent with properties similar to N-methylpyrrolidone and sulfolane, both of which are traditionally obtained from fossil-based resources. HDO, traditionally derived from benzene, is used in the production of polymers and coatings. The current state-of-the-art in LGO production uses a vacuum pyrolysis approach, leading to a complex mixture of compounds from which LGO must be isolated. In contrast, we demonstrate that tetrahydrofuran can be used to produce LGO at low temperatures in the liquid phase. Importantly, the yield of LGO obtained using this liquid-phase process is higher than those obtained by catalytic pyrolysis routes, and the crude product solution is much less complex than pyrolysis products, leading to simpler LGO recovery strategies.
|
Introduction
Biomass is one of the few renewable sources of carbon. Lignocellulose is the most abundant source of biomass.1–4 Furans produced by acid hydrolysis of saccharides (i.e., 5-hydroxymethylfurfural (HMF) and furfural) are one of the most promising chemical feedstocks that can be produced from biomass.5–12 Cellulose can also be depolymerized to form anhydro-sugars either by pyrolysis13–15 or dehydration under anhydrous conditions.16–19 Levoglucosenone (1,6-anhydro-3,4-dideoxy-β-D-pyranosen-2-one, LGO) is an anhydro-sugar that contains a double bond conjugated with a ketone as well as a protected aldehyde and two protected hydroxyl groups.20,21 Its unique structure and potential as a chiral building block have made it an attractive platform molecule, as shown in Scheme 1. During the last decade, this substrate has been used as building block22,23 for the synthesis of a wide variety of high value pharmaceuticals (e.g., (+)-chloriolide, which is a macrolide antibiotic,24 and ras activition inhibitors, which are anticancer drugs25). The hydrogenated form of LGO, dihydrolevoglucosenone (Cyrene®), is useful as a bio-based polar, aprotic solvent with properties similar to NMP, DMF, and sulfolane.26 5-Hydroxymethyldihydrofuranone, a precursor for nucleic acids, can also be produced from dihydrolevoglucosenone.27 Importantly, 1,6-hexanediol, widely used in the polymer industry, can also be produced in 69% molar yield by the aqueous-phase hydrogenation of LGO using Pt/C and Pt/W/TiO2.28–30
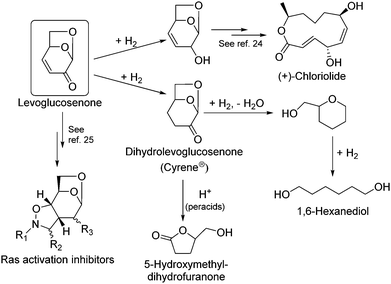 |
| Scheme 1 Potential uses of levoglucosenone. | |
LGO has traditionally been produced in low yields by catalytic fast pyrolysis of biomass, and many attempts have been made to improve the LGO yield using pyrolysis technologies.17,31–39 Notably, Dobele and coworkers pre-treated wood with phosphoric acid prior to pyrolysis, with LGO making up 30% of the total peak area of the detectable products at 375 °C.38 Zhang and coworkers explored sulfated zirconia, a solid superacid, as a means to improve the yield of LGO, but their best carbon yield was only 8.4%.37 These low yields make it difficult to recover LGO from bio oil and have so far limited the large-scale exploitation of LGO.
Solvent-assisted pyrolysis leads to enhanced yields by inhibiting the polymerization of levoglucosan (LGA) and LGO, which are the primary cellulose dehydration products. For example, Kudo et al. used a sulfonate ionic liquid ([EMIM]CH3C6H4SO3) as a solvent and a catalyst for LGO production from saccharides by pyrolysis, and they achieved an LGO yield of 28.9 wt%.18 Kawamoto et al. used a complicated vacuum pyrolysis approach to convert cellulose to LGO using sulfolane with sulfuric acid or phosphoric acid (0.1–1.0 wt% acid concentration) as catalysts. The molar yields of LGO, furfural, and HMF were as high as 42.2%, 26.9%, and 8.8%, respectively when the pressure was reduced to 0.1 atm.16 In recent work, we have shown that monophasic systems containing 90 wt% γ-valerolactone (GVL) and 10 wt% water can be used to selectively produce levulinic acid from cellulose with yields close to 70% using Amberlyst-70 as the catalyst.40 We have also used GVL with H2SO4 as the catalyst to break down cellulose and hemicellulose into their constituent sugars, and obtained yields of 70–90%.41 In the same vein, we have reported that cellulose can be effectively converted to HMF in tetrahydrofuran (THF) under mild reaction conditions, obtaining an HMF yield of 44%.42 In related work, Wyman et al. have shown that THF, when used as a co-solvent with water, leads to an increase in the yield of HMF, furfural and levulinic acid43,44 or the yield of monosugars45 obtained during biomass hydrolysis. Notably, this work suggests that THF can be recycled when used with inorganic acids such as H2SO4.
Herein, we demonstrate an approach to easily produce LGO with high yield from cellulose under mild reaction conditions using polar, aprotic solvents such as THF and GVL. The yield of LGO is higher than has been obtained using ionic liquids or sulfolane. Moreover, HMF and furfural are the only volatile by-products, and these can be recovered by distillation. Finally, we show that the product distribution can be adjusted by optimizing the reaction conditions.
Experimental
Reaction studies
Reactions were performed in a 100 mL Hastelloy (C-276) autoclave (Parr Instrument Company, series 4560). The vessel and head were dried overnight at 70 °C to remove residual water prior to each reaction. Solutions of cellulose (Avicel® PH-101, moisture content ca. 3 wt%), cellobiose (Sigma Aldrich, purity ≥98%), glucose (Sigma Aldrich, anhydrous), and LGA (Sigma Aldrich, purity 99%), THF (Sigma Aldrich, anhydrous, 99.9%, inhibitor free), and sulfuric acid (Fisher Chemical, A300-500) were sealed in the autoclave, at which point the vessel was purged five times with helium (Airgas). The vessel was then heated to the desired reaction temperature and pressurized to a final pressure of 6.9 MPa. Zero time was defined as the point at which the heating was started, except where noted. The stirring rate was maintained at 600 rpm. The temperature and stirring were controlled by a Parr 4848 Controller. Samples (about 1 mL) were periodically withdrawn through a dip tube. The reactor was repressurized with helium after withdrawing each sample. The samples were immediately quenched in an ice water bath and filtered with a 0.2 μm syringe filter (IC Millex®-LG). Samples were diluted twice with water prior to analysis.
Analytical methods
LGO (standards purchased from GlycoSyn, purity 98%) and HMF (standards purchased from Sigma Aldrich, purity ≥98%) were analyzed using a gas chromatograph (Shimadzu, GC-2010 equipped with a flame ionization detector and a Restek RTX-VMS capillary column). The injection port and the detector were held at 240 °C. The column flow rate was 0.43 mL min−1 with a He carrier gas. The GC oven temperature was initially held at 40 °C for 5 min, ramped to 240 °C at 7.5 °C min−1, and kept at 240 °C for 15 min. LGA, glucose and furfural were analyzed using a high-performance liquid chromatograph (HPLC; Shimadzu, LC-20AT) equipped with UV (UV-vis; SPD-20AV) and RI (RID-10A) detectors. Separation was achieved using a Biorad Aminex HPX-87H column at 30 °C with 5 mM H2SO4 as the mobile phase, flowing at a rate of 0.6 mL min−1. For each analysis, the injection volume was 1 μL.
All yields were calculated as follows, where detectable products consisted of glucose, levoglucosan, levoglucosenone, HMF, and furfural:
Results and discussion
Product identification and quantification
The main detectable products of the reaction are glucose, LGA, LGO, furfural, HMF, and levulinic and formic acids. Gas chromatography (GC) is typically used to analyze many of these compounds when studying catalytic fast pyrolysis, although glucose and LGA must be analyzed using liquid phase techniques such as high performance liquid chromatography (HPLC). The Biorad Aminex HPX-87H organic acid column that is typically used in HPLC analyses of biomass hydrolyzates cannot resolve LGO from HMF. This lack of resolution is not typically a problem in systems where hydrolysis is the target reaction, because little LGO is formed except under anhydrous conditions (discussed below). Fig. 1 shows the analysis of a standard sample containing glucose, LGA, LGO, furfural, HMF, and levulinic and formic acids. The HPLC chromatogram contains one fewer peak than the total number of compounds because LGO and HMF elute with the same retention time. In contrast, analysis by GC resolves LGO from HMF, but glucose, LGA, and formic acid are not detected due to the decomposition of glucose and LGA in the injector and the poor sensitivity of formic acid. Therefore, reactions performed in the liquid phase need to be analyzed by both GC and HPLC to adequately quantify all of the potential products.
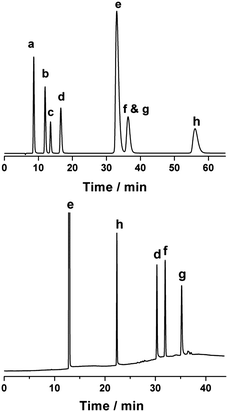 |
| Fig. 1 HPLC (top) and GC (bottom) chromatograms of the products produced from cellulose dehydration in THF. (a) Glucose, (b) LGA, (c) formic acid, (d) levulinic acid, (e) THF, (f) LGO, (g) HMF, (h) furfural. | |
Solvent selection
We have previously shown that the use of polar, aprotic solvents is a promising method for converting cellulose to HMF.42 However, as discussed above, the presence of LGO can convolute the results obtained by the standard analytical method using only HPLC. Consequently, we have used both HPLC and GC to reanalyze several samples from our previous work on cellulose decomposition with dilute sulfuric acid in different solvents (presented in Table 1). It is important to note that while some LGO was produced during reactions run under anhydrous conditions, the overall trend in HMF yield with changing solvent is the same as previously reported. Further, as discussed below, only small amounts of LGO are formed when water is present, and when analyzing the products by GC, using the optimal reaction conditions, we obtain the same yield of HMF as reported previously.42 Thus, modifying the analytical method does not change the conclusions of our previous work.
Table 1 Dehydration of cellulose to LGO and HMF in different solventsa
Reaction time [min] |
LGO yield [%] |
THF |
GVL |
Ethyl acetateb |
Acetoneb |
Ethanolb |
Waterb |
Reaction conditions: 50 g L−1 cellulose, 60 mL total volume, 5 mM sulfuric acid, 170 °C.
Reanalyzed from ref. 42.
Values in parenthesis were reported in ref. 42.
|
30 |
6 |
10 |
2 |
2 |
0 |
0 |
60 |
8 |
15 |
4 |
4 |
0 |
0 |
Reaction time [min] |
HMF yieldb,c [%] |
THF |
GVL |
Ethyl acetateb |
Acetoneb |
Ethanolb |
Waterb |
30 |
1 (8) |
2 (7) |
0 (1) |
2 (9) |
0 (0) |
0 (0) |
60 |
1 (14) |
4 (10) |
0 (1) |
3 (14) |
0 (1) |
0 (0) |
No LGO was produced when using water or ethanol, both of which are protic solvents. The use of acetone and ethyl acetate led to 2–3% yields of LGO, while the use of THF and GVL led to the highest yields of LGO, with increasing yields obtained at longer reaction times. Consequently, we examined the yield of LGO as a function of reaction time using both GVL and THF as solvents at both 170 °C and 190 °C (see Fig. 2). Although higher yields of LGO from cellulose are initially obtained in GVL, the yield of LGO in THF increased continuously, eventually surpassing the maximum yield obtained in GVL. Importantly, LGO appears to be stable in THF at reaction times of up to 6 hours, whereas LGO degrades in GVL. At 190 °C, higher yields of LGO were obtained when using THF than when using GVL. As shown in Table 2, the initial rates of LGO and LGA production were higher in GVL than in THF. Notably, the rate of HMF production relative to the rate of LGO production in GVL is nearly twice that in THF at both temperatures, suggesting that GVL promotes the formation of HMF.
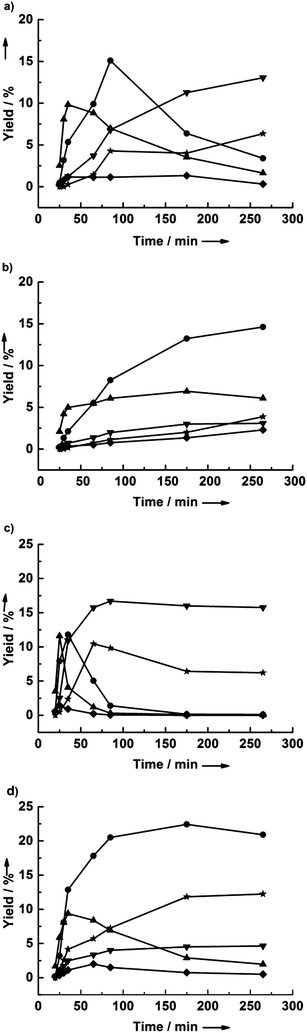 |
| Fig. 2 Dehydration of cellulose in: (a) GVL at 170 °C, (b) THF at 170 °C, (c) GVL at 190 °C, and (d) THF at 190 °C. LGO (●), LGA (▲), glucose (◆), HMF (★), furfural (▼). Reaction conditions: 50 g L−1 cellulose, 60 mL total volume, 5 mM (a, b) or 7.5 mM (c, d) sulfuric acid. | |
Table 2 Initial LGO, LGA, and HMF turnover frequencies (TOFs)a
Solvent |
Temp. [°C] |
Acid [mM] |
Initial TOFb [ks−1] |
HMF : LGO |
LGO |
LGA |
HMF |
Assuming one proton per mole of H2SO4. Reaction conditions: 50 g L−1 cellulose, 60 mL total reaction volume.
Uncertainties correspond to the standard error of the slope.
Not detected. The LGO production rate is sufficiently high at this condition that the maximum LGA concentration was achieved prior to taking the first sample.
|
THF |
170 |
5 |
0.67 ± 0.1 |
0.45 ± 0.2 |
0.09 ± 0.01 |
0.14 |
THF |
190 |
7.5 |
1.5 ± 0.4 |
1.2 ± 0.2 |
0.28 ± 0.1 |
0.19 |
GVL |
170 |
5 |
1.2 ± 0.1 |
3.7 ± 1 |
0.35 ± 0.1 |
0.30 |
GVL |
190 |
7.5 |
3.8 ± 0.7 |
n.d.c |
1.1 ± 0.1 |
0.29 |
Effect of water content
Fig. 3 shows the effect of water content on the dehydration of cellulose in THF at 190 °C. In pure THF, cellulose is converted to LGA, and the LGA is subsequently converted to LGO. The maximum yield of LGO was 47%, after 4 hours of reaction. Notably, this yield is higher than many reported yields of HMF from cellulose. Only a trace amount of glucose was detected when using pure solvents, and the water required for hydrolysis likely comes from the dehydration of LGA. The conversion of cellulose to glucose was dramatically enhanced by the addition of 2.7 wt% water. LGA and glucose, obtained from cellulose dehydration and hydrolysis, respectively, were both detected at short reaction times (30 minutes). The highest yields of LGA and glucose were 33 and 19%, respectively. Notably, the yield of LGO with 2.7% water decreased by more than half compared to that obtained in pure THF (i.e., an LGO yield of 21%). The HMF yield increased fivefold with the addition of 2.7 wt% water to the THF, eventually reaching 25% yield. Cellulose dehydration to LGO was completely inhibited in the presence of 11 wt% water, while cellulose hydrolysis to glucose was promoted. The glucose yield went through a maximum, suggesting that it is converted to HMF and humins, where formation of the latter was indicated by insoluble precipitates in the reactor. Interestingly, the yield of furfural remained essentially constant in our reactions regardless of the presence of water and despite the increase in the HMF yield with increasing water content.
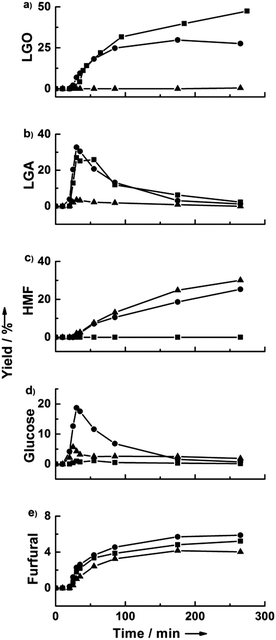 |
| Fig. 3 Effect of water content on cellulose dehydration. (a) LGO, (b) LGA, (c) HMF, (d) glucose, (e) furfural. Pure THF (■), 2.7 wt% water in THF (●), 11.6 wt% water in THF (▲). Reaction conditions: 1 wt% cellulose, 7.5 mM sulfuric acid, 190 °C, 60 mL total reaction volume. | |
Effect of cellulose loading and acid concentration
We examined the effect of cellulose loading ranging from 1 to 5 wt% and sulfuric acid concentration. As shown in Table 3, the maximum LGO yield decreases from 47% to 21% with increasing cellulose loading. The total carbon yield and the LGA yield also decreased with increasing cellulose loading. The yield of glucose was always low due to the absence of water in these reactions. In contrast, the yield and concentration of HMF increased with increasing cellulose loading, while the yield of furfural remained constant. At longer reaction times, the yields of all compounds except furfural decreased. Table 3 also shows that increasing the acid concentration from 7.5 to 20 mM has a minimal effect on the LGO yield for the conversion of 5 wt% cellulose.
Table 3 Effect of cellulose loading and sulfuric acid concentration on the product distribution
Cellulose [wt%] |
Sulfuric acid [mM] |
Timea [h] |
LGO yieldb [%] |
LGO |
LGA |
HMF |
Glucose |
Furfural |
Total |
For these reactions, t = 0 was defined as the point at which the reactor reached the reaction temperature.
Reaction conditions: 60 mL total volume, 190 °C.
|
1 |
7.5 |
1 |
32 |
12 |
0 |
1 |
4 |
49 |
4 |
47 |
2 |
0 |
0 |
5 |
54 |
|
3 |
7.5 |
1 |
28 |
9 |
6 |
1 |
4 |
48 |
4 |
31 |
3 |
9 |
0 |
5 |
48 |
|
5 |
7.5 |
1 |
21 |
7 |
7 |
1 |
4 |
40 |
4 |
21 |
2 |
12 |
0 |
5 |
40 |
6 |
21 |
1 |
13 |
0 |
5 |
40 |
10 |
18 |
1 |
12 |
0 |
5 |
36 |
|
5 |
20 |
1 |
20 |
1 |
14 |
0 |
5 |
40 |
4 |
13 |
0 |
10 |
0 |
5 |
28 |
Effect of temperature
The effect of reaction temperature is shown in Table 4. A maximum LGO yield of 51% was obtained after 30 min at 210 °C. A maximum LGO yield of 47% was obtained at 10 minutes at a temperature of 230 °C. A similar improvement in yield with increasing temperature was observed at a 3% cellulose loading. Notably, the reaction solution contained only LGO, HMF, and furfural when the reaction was stopped.
Table 4 Effect of temperature on the product distribution
Cellulose [wt%] |
Temperature [°C] |
Timea [min] |
Yieldb [%] |
LGO |
LGA |
HMF |
Glucose |
Furfural |
Total |
For these reactions, t = 0 was defined as the point at which the reactor reached the reaction temperature.
Reaction conditions: 60 mL total volume, 20 mM sulfuric acid.
|
1 |
190 |
10 |
29 |
17 |
0 |
1 |
3 |
50 |
30 |
39 |
6 |
2 |
0 |
4 |
51 |
60 |
43 |
2 |
2 |
0 |
4 |
51 |
|
1 |
210 |
10 |
40 |
8 |
0 |
0 |
4 |
52 |
30 |
51 |
0 |
1 |
0 |
5 |
57 |
60 |
50 |
0 |
2 |
0 |
6 |
58 |
|
1 |
230 |
10 |
47 |
0 |
1 |
0 |
5 |
53 |
30 |
40 |
0 |
3 |
0 |
6 |
49 |
60 |
30 |
0 |
5 |
0 |
6 |
41 |
|
3 |
210 |
10 |
25 |
7 |
0 |
1 |
4 |
37 |
30 |
33 |
1 |
1 |
0 |
5 |
40 |
60 |
32 |
0 |
1 |
0 |
6 |
39 |
The stability of LGO in THF
While studying the effect of feedstock loading, we observed that the LGO yield decreased with time (see Table 3). To confirm this result, we performed a reaction for 44.5 h at 190 °C using 1 wt% cellulose in THF with 7.5 mM sulfuric acid as a catalyst. As shown in Fig. 4, the yield of LGO reached a maximum at 4.5 h, after which the yield decreased gradually to one third of the maximum value. As noted above, LGA is a primary product that is consumed rapidly, and no glucose was detected because the reaction was run in the absence of water. The HMF and furfural yields remained constant over the same time period used in our previous study.42
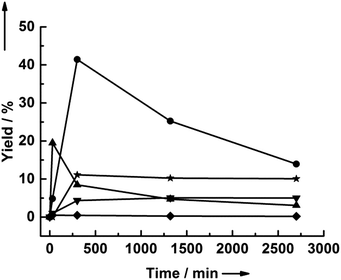 |
| Fig. 4 Time-course of cellulose dehydration for 44 hours. LGO (●), LGA (▲), glucose (◆), HMF (★), furfural (▼). Reaction conditions: 1 wt% cellulose, 7.5 mM sulfuric acid, 190 °C, 60 mL total reaction volume. | |
Elucidation of the reaction network
Fig. 5 shows the results of the dehydration of cellobiose, LGA, and glucose in pure THF and 2.7 wt% water in THF. In THF, the LGA is rapidly converted to primarily LGO in yields up to 49% (see Fig. 5a). The other detectable product was HMF, which reached a 6% yield. No glucose was detected for LGA conversion in pure THF, suggesting that HMF may be derived by the isomerization of LGO. In contrast, the yield of LGO was only 22% in the presence of 2.7 wt% water, and the HMF yield increased to 28% (see Fig. 5b). Glucose was also observed in this reaction and passed through a maximum at about 25 minutes. This result indicates that LGA can be rehydrated under these conditions. It is difficult to discern whether the improvement in HMF yield is primarily due to glucose conversion or due to water-assisted LGO isomerization.
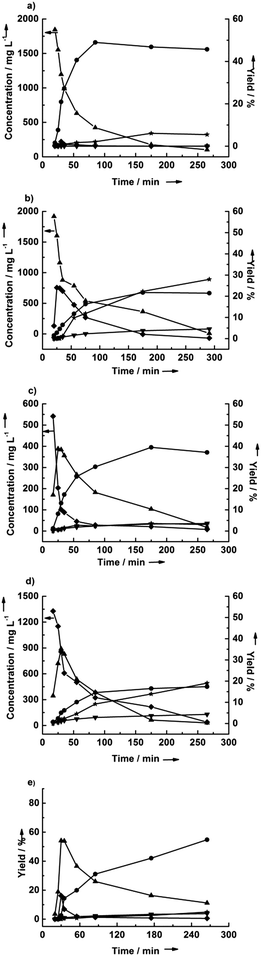 |
| Fig. 5 Conversion of LGA, glucose, and cellobiose in THF and water. (a) LGA in THF, (b) LGA in 2.7% water in THF, (c) glucose in THF, (d) glucose in 2.7% water in THF, (e) cellobiose in THF. Key: LGO (●), LGA (▲), glucose (◆), HMF (★), and furfural (▼). Reaction conditions: 0.4 wt% LGA, 0.7 wt% glucose, and 1.2 wt% cellobiose, 7.5 mM sulfuric acid, 190 °C, 60 mL total reaction volume. | |
A similar result was obtained during glucose dehydration (Fig. 5c and d). LGO was formed at 40% yield in the absence of water, with LGA as an intermediate. The presence of water increased the HMF yield from 4% to 19%. Interestingly, the furfural yield was 3–4% regardless of the presence of water, suggesting that furfural may be produced directly from glucose, as has been reported previously.46
Cellobiose showed the same dehydration behavior as cellulose. Notably, Assary and Curtiss calculated the activation barrier for cellobiose conversion to LGO to be 59 kcal mol−1, in good agreement with the experimentally measured activation energy required for the formation of activated cellulose (58 kcal mol−1),18,47 which should translate to similar yields of LGO from each. However, the maximum yields of glucose, LGA, and LGO were higher when starting from cellobiose than when starting from cellulose, which may be due to the recalcitrance of crystalline cellulose.
Also notable is the production of furfural in the presence of water, while no furfural was observed in the absence of water (see Fig. 5a and b). Moreover, furfural production is independent of water concentration for reactions starting from cellulose, as shown in Fig. 3e. However, if the water-assisted conversion of LGO to furfural were a significant reaction, one would expect the furfural production from cellulose to increase with increasing water content. Therefore, based on the results presented in Fig. 3e and Fig. 5a and b, we suggest that the conversion of LGO to furfural does not readily occur in THF, in contrast to Kawamoto's observations for the reaction in sulfolane.16 Indeed, the choice of solvent appears to be important, because we have observed that THF inhibits the conversion of LGO to HMF (see Fig. 2 and 5), GVL favors the isomerization of LGO to HMF (see Fig. 2), and Kawamoto and coworkers observed that sulfolane favors the conversion of LGO to furfural.16
From the above results, we propose the reaction network shown in Scheme 2 for the dehydration of cellulose to LGO in THF. There are two competitive routes for cellulose decomposition. One route is the depolymerization reaction that occurs in the absence of water, which is analogous to the pyrolytic depolymerization of cellulose to LGA previously illustrated by our group48 and other research groups.49–53 In this scheme, LGA is the primary product of cellulose depolymerization, which is then further dehydrated to yield LGO. The transformation of LGA to LGO can occur by two pathways (see Scheme 3). The commonly proposed mechanism involves the sequential dehydration of LGA, as suggested by Broido.20 Importantly, Broido suggested that isolevoglucosenone could also be produced from LGA, although they did not detect this compound. Assary and Curtiss computed the thermodynamics of both pathways, which may suggest that both species could be formed, although the path to LGO is slightly more direct than that to iso-LGO. The second mechanism, presented by Shafizadeh et al., involves 1,4:3,6-dianhydro-a-D-glucopyranose (DGP) as an intermediate between LGA and LGO.54 Such a mechanism successfully predicts the absence of iso-LGO in the pyrolysates and in our reactions, although it may be difficult to distinguish iso-LGO from LGO using GC analysis. We do not detect the presence of DGP or of a singly-dehydrated LGA species, so we cannot distinguish which mechanism is operative under our reaction conditions.
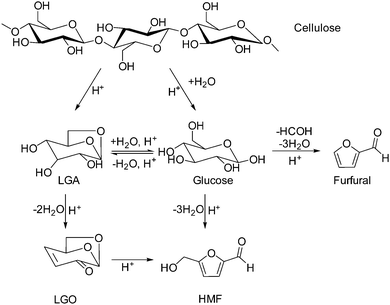 |
| Scheme 2 The proposed reaction network for converting cellulose to LGO in THF. | |
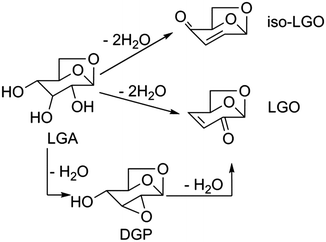 |
| Scheme 3 Potential conversion pathways from LGA to LGO. | |
The second route for cellulose conversion is the conventional cellulose hydrolysis pathway. As expected, glucose is the primary product of cellulose hydrolysis in aqueous medium, and it can quickly undergo isomerization and dehydration with acid catalysts to yield HMF.46 LGA and glucose may also be interconverted in the presence of water. Notably, the interconversion between these routes is governed by the addition or removal of water, so the final product distribution can be tuned by adjusting the water content in the reaction medium. Without water, the main products are LGO and LGA, with small amounts of HMF and furfural also being formed. However, cellulose dehydration was inhibited completely in the presence of small amounts of water, and no anhydrosugars were observed. HMF and furfural were the only products of these reactions and the yields were observed to be higher in the presence of water than in the absence of water. Small amounts of levulinic acid were also formed in the presence of water, as were insoluble precipitates (i.e., humins).
Conclusions
We have presented an approach to produce LGO and LGA in high yields from cellulose under mild reaction conditions using polar, aprotic solvents. To our knowledge, this is the highest LGO yield to be reported. The final product distribution can be controlled by the addition of water to the reaction medium. HMF gradually replaces LGO as the main product with increasing water content in the solvent. LGO is a promising building block that can be used to make a variety of renewable chemicals from biomass.
Acknowledgements
The authors acknowledge financial support provided by the Priority Academic Program Development of Jiangsu Higher Education Institutions (PAPD) and Jiangsu Government Scholarship for Overseas Studies. T.J.S. acknowledges support from the National Science Foundation Graduate Research Fellowship Program under Grant No. DGE-1256259. Any opinions, findings, and conclusions or recommendations expressed in this material are those of the authors and do not necessarily reflect the views of the National Science Foundation.
Notes and references
- D. M. Alonso, J. Q. Bond and J. A. Dumesic, Green Chem., 2010, 12, 1493–1513 RSC.
- C.-H. Zhou, X. Xia, C.-X. Lin, D.-S. Tong and J. Beltramini, Chem. Soc. Rev., 2011, 40, 5588–5617 RSC.
- S. G. Wettstein, D. M. Alonso, E. I. Gürbüz and J. A. Dumesic, Curr. Opin. Chem. Eng., 2012, 1, 218–224 CrossRef CAS PubMed.
- M. J. Climent, A. Corma and S. Iborra, Green Chem., 2014, 16, 516–547 RSC.
- P. Gallezot, Chem. Soc. Rev., 2012, 41, 1538–1558 RSC.
- R. Karinen, K. Vilonen and M. Niemela, ChemSusChem, 2011, 4, 1002–1016 CrossRef CAS PubMed.
- A. A. Rosatella, S. P. Simeonov, R. F. M. Frade and C. A. M. Afonso, Green Chem., 2011, 13, 754–793 RSC.
- B. Saha and M. M. Abu-Omar, Green Chem., 2014, 16, 24–38 RSC.
- T. Stahlberg, W. Fu, J. M. Woodley and A. Riisager, ChemSusChem, 2011, 4, 451–458 CrossRef CAS PubMed.
- R.-J. van Putten, J. C. van der Waal, E. de Jong, C. B. Rasrendra, H. J. Heeres and J. G. de Vries, Chem. Rev., 2013, 113, 1499–1597 CrossRef CAS PubMed.
- T. Wang, M. W. Nolte and B. H. Shanks, Green Chem., 2014, 16, 548–572 RSC.
- M. E. Zakrzewska, E. Bogel-Lukasik and R. Bogel-Lukasik, Chem. Rev., 2011, 111, 397–417 CrossRef CAS PubMed.
- Y.-C. Lin, J. Cho, G. A. Tompsett, P. R. Westmoreland and G. W. Huber, J. Phys. Chem. C, 2009, 113, 20097–20107 CAS.
- N. Kuzhiyil, D. Dalluge, X. Bai, K. H. Kim and R. C. Brown, ChemSusChem, 2012, 5, 2228–2236 CrossRef CAS PubMed.
- X. Gong, Y. Yu, X. Gao, Y. Qiao, M. Xu and H. Wu, Energy Fuels, 2014, 28, 5204–5211 CrossRef CAS.
- H. Kawamoto, S. Saito, W. Hatanaka and S. Saka, J. Wood Sci., 2007, 53, 127–133 CrossRef CAS.
- S. Kudo, Z. Zhou, K. Norinaga and J.-i. Hayashi, Green Chem., 2011, 13, 3306–3311 RSC.
- S. Kudo, Z. Zhou, K. Yamasaki, K. Norinaga and J.-i. Hayashi, Catalysts, 2013, 3, 757–773 CrossRef PubMed.
- X. Bai, R. C. Brown, J. Fu, B. H. Shanks and M. Kieffer, Energy Fuels, 2014, 28, 1111–1120 CrossRef CAS.
- Y. Halpern, R. Riffer and A. Broido, J. Org. Chem., 1973, 38, 204–209 CrossRef CAS.
- M. S. Miftakhov, F. A. Valeev and I. N. Gaisina, Usp. Khim., 1994, 63, 922–936 CAS.
- A. M. Sarotti, M. M. Zanardi, R. A. Spanevello and A. G. Suarez, Curr. Org. Synth., 2012, 9, 439–459 CrossRef CAS.
- V. Corne, M. C. Botta, E. D. V. Giordano, G. F. Giri, D. F. Llompart, H. D. Biava, A. M. Sarotti, M. I. Mangione, E. G. Mata, A. G. Suarez and R. A. Spanevello, Pure Appl. Chem., 2013, 85, 1683–1692 CrossRef CAS PubMed.
- M. Ostermeier and R. Schobert, J. Org. Chem., 2014, 79, 4038–4042 CrossRef CAS PubMed.
- C. Muller, M. Frau, D. Ballinari, S. Colombo, A. Bitto, E. Martegani, C. Airoldi, A. S. van Neuren, M. Stein, J. Weiser, C. Battistini and F. Peri, ChemMedChem, 2009, 4, 524–528 CrossRef PubMed.
- J. Sherwood, M. De Bruyn, A. Constantinou, L. Moity, C. R. McElroy, T. J. Farmer, T. Duncan, W. Raverty, A. J. Hunt and J. H. Clark, Chem. Commun., 2014, 50, 9650–9652 RSC.
-
K. Koseki, T. Ebata, H. Kawakami, H. Matsushita, K. Itoh and Y. Naoi, US5112994 A, 1992.
-
A. M. Allgeier, N. Desilva, E. Korovessi, C. Menning, J. C. Ritter and S. K. Sengupta, WO2013101980 A1, 2013.
-
A. M. Allgeier, N. Desilva, E. Korovessi, C. Menning, J. C. Ritter, S. K. Sengupta and C. S. Stauffer, US20130172578 A1, 2013.
-
A. M. Allgeier, J. C. Ritter and S. K. Sengupta, US20130231505 A1, 2013.
- M. Miura, H. Kaga, T. Yoshida and K. Ando, J. Wood Sci., 2001, 47, 502–506 CrossRef CAS.
- G. Dobele, T. Dizhbite, G. Rossinskaja, G. Telysheva, D. Mier, S. Radtke and O. Faix, J. Anal. Appl. Pyrolysis, 2003, 68–69, 197–211 CrossRef CAS.
- A. M. Sarotti, R. A. Spanevello and A. G. Suarez, Green Chem., 2007, 9, 1137–1140 RSC.
-
J. A. Marshall, An improved preparation of levoglucosenone from cellulose, Iowa State University, 2008 Search PubMed.
- Q. Lu, X.-c. Yang, C.-q. Dong, Z.-f. Zhang, X.-m. Zhang and X.-f. Zhu, J. Anal. Appl. Pyrolysis, 2011, 92, 430–438 CrossRef CAS PubMed.
- Q. Lu, X.-M. Zhang, Z.-B. Zhang, Y. Zhang, X.-F. Zhu and C.-Q. Dong, BioResources, 2012, 7, 2820–2834 CrossRef CAS PubMed.
- X.-w. Sui, Z. Wang, B. Liao, Y. Zhang and Q.-x. Guo, Bioresour. Technol., 2012, 103, 466–469 CrossRef CAS PubMed.
- J. Zandersons, A. Zhurinsh, G. Dobele, V. Jurkjane, J. Rizhikovs, B. Spince and A. Pazhe, J. Anal. Appl. Pyrolysis, 2013, 103, 222–226 CrossRef CAS PubMed.
- X. Wei, Z. Wang, Y. Wu, Z. Yu, J. Jin and K. Wu, J. Anal. Appl. Pyrolysis, 2014, 107, 150–154 CrossRef CAS PubMed.
- D. M. Alonso, S. G. Wettstein and J. A. Dumesic, Green Chem., 2013, 15, 584–595 RSC.
- J. S. Luterbacher, J. M. Rand, D. M. Alonso, J. Han, J. T. Youngquist, C. T. Maravelias, B. F. Pfleger and J. A. Dumesic, Science, 2014, 343, 277–280 CrossRef CAS PubMed.
- R. Weingarten, A. Rodriguez-Beuerman, F. Cao, J. S. Luterbacher, D. M. Alonso, J. A. Dumesic and G. W. Huber, ChemCatChem, 2014, 6, 2229–2234 CrossRef CAS PubMed.
- C. M. Cai, T. Zhang, R. Kumar and C. E. Wyman, Green Chem., 2013, 15, 3140–3145 RSC.
- C. M. Cai, N. Nagane, R. Kumar and C. E. Wyman, Green Chem., 2014, 16, 3819–3829 RSC.
- T. Y. Nguyen, C. M. Cai, R. Kumar and C. E. Wyman, ChemSusChem, 2015 DOI:10.1002/cssc.201403045.
- E. I. Guerbuez, J. M. R. Gallo, D. M. Alonso, S. G. Wettstein, W. Y. Lim and J. A. Dumesic, Angew. Chem., Int. Ed., 2013, 52, 1270–1274 CrossRef CAS PubMed.
- R. S. Assary and L. A. Curtiss, ChemCatChem, 2012, 4, 200–205 CrossRef CAS PubMed.
- Y.-C. Lin, J. Cho, G. A. Tompsett, P. R. Westmoreland and G. W. Huber, J. Phys. Chem. C, 2009, 113, 20097–20107 CAS.
- Z. Y. Luo, S. R. Wang, Y. F. Liao and K. F. Cen, Ind. Eng. Chem. Res., 2004, 43, 5605–5610 CrossRef CAS.
- D. K. Shen and S. Gu, Bioresour. Technol., 2009, 100, 6496–6504 CrossRef CAS PubMed.
- P. R. Patwardhan, J. A. Satrio, R. C. Brown and B. H. Shanks, Bioresour. Technol., 2010, 101, 4646–4655 CrossRef CAS PubMed.
- M. S. Mettler, S. H. Mushrif, A. D. Paulsen, A. D. Javadekar, D. G. Vlachos and P. J. Dauenhauer, Energy Environ. Sci., 2012, 5, 5414–5424 CAS.
- M. S. Mettler, A. D. Paulsen, D. G. Vlachos and P. J. Dauenhauer, Energy Environ. Sci., 2012, 5, 7864–7868 CAS.
- F. Shafizadeh, R. H. Furneaux and T. T. Stevenson, Carbohydr. Res., 1979, 71, 169–192 CrossRef CAS.
|
This journal is © The Royal Society of Chemistry 2015 |
Click here to see how this site uses Cookies. View our privacy policy here.