DOI:
10.1039/C4CC09268F
(Feature Article)
Chem. Commun., 2015,
51, 3290-3301
Asymmetric catalysis activated by visible light
Received
20th November 2014
, Accepted 2nd January 2015
First published on 9th January 2015
Abstract
Visible light driven organic chemistry has sparked much excitement over the last several years. This review summarizes recent progress in combining visible light activation with asymmetric catalysis, processes that are either mediated by photoinduced electron or energy transfer. The tasks of photoactivation and asymmetric catalysis are typically accomplished by dual catalyst systems but several recent reports demonstrate that they can also be effectively executed by single catalysts. Beyond the discovery of novel asymmetric transformations under mild reaction conditions, this contemporary area of organic chemistry holds promise for the development of economical and environmentally friendly methods for the asymmetric synthesis of chiral compounds.
Introduction
Asymmetric catalysis provides vast opportunities for an economical access to chiral compounds in enantiomerically pure form.1 Sun light, on the other hand, is considered an inexpensive, abundant, and environmentally benign form of energy and the last several years have witnessed a surge in studies exploiting visible light for activating chemical transformations.2 Interfacing visible light activated photochemistry with asymmetric catalysis therefore provides new opportunities for the development of synthetic methods for the efficient and green synthesis of non-racemic chiral molecules. Beyond cost and environmental factors, compared to the well studied UV light induction of chemical reactions,3 the activation by visible light circumvents problems associated with UV photodegradation and obviates the need for specialized equipment. In a typical experimental setup, visible light in form of sunlight, a LED light source, or a standard household lamp, is absorbed by a photosensitizer (PS) which then triggers an energy or electron transfer process (Fig. 1). In the latter case, the photoactivated sensitizer (PS*) is a stronger reducing and oxidizing agent compared to the ground state, so that photoinduced redox chemistry generates reactive radical cation or radical anion intermediates that possess unusual reactivities under surprisingly mild reaction conditions due to the low steady state concentration of the photoactivated redox sensitizer in combination with the fact that organic compounds often do not absorb visible light.4 In this context, activation by single electron transfer (SET) can be considered a complement to the well established and exploited activation by proton transfer.5 Whereas photoredox chemistry has experienced a renaissance over the last several years, the control of absolute stereochemistry in photoredox reactions is still in its infancy. This review therefore summarizes and discusses recent progress in merging visible light activated photochemistry with asymmetric catalysis and highlights underlying concepts.
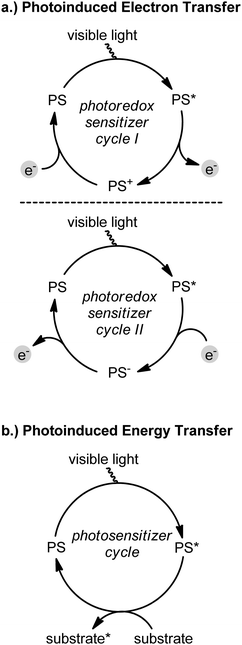 |
| Fig. 1 Induction of chemical processes by visible light activated photosensitizers (PS). (a) The photoactivated redox sensitizer is a stronger reducing agent (photoredox cycle I) and oxidizing agent (photoredox cycle II) than the ground state. Note that either PS* or PS+ and PS* or PS− can serve as the actual oxidizing and reducing agents, respectively, for the substrates. As a consequence, both photoredox cycles are capable of inducing the initial oxidation or reduction of the redox active substrates. (b) Photoinduced energy transfer either occurs from a singlet or triplet excited state of the photosensitizer. | |
Photoinduced electron transfer: redox neutral reactions
Merging asymmetric enamine catalysis with photoredox catalysis
In 2008, MacMillan’s group reported the first example of merging visible light induced photoredox catalysis with asymmetric organocatalysis and the generality of this strategy was established in subsequent publications (Table 1).6–9 Accordingly, the enantioselective intermolecular α-alkylation of aldehydes with electron deficient alkylating agents was accomplished using a combination out of transition metal photoredox catalysts and the imidazolidinone organocatalysts 1 or 2. Work by Zeitler,10 König,11 and Pericàs12 revealed that organic dyes and inorganic semiconductors are also promising photosensitizers in this system. Mechanistically, it is believed that a photoredox catalytic cycle results in the reductive, heterolytic cleavage of the electrophiles, such as acceptor-substituted benzyl bromides, phenacyl bromides, or perfluoralkyl iodides, to afford electron deficient alkyl radicals which rapidly react with the electron rich double bond of chiral intermediate enamines in a stereocontrolled fashion (Fig. 2).13 Thereby generated α-amino radicals are prone to oxidation and are hydrolyzed after single electron oxidation to iminium ions. The electron either directly flows back into the photoredox cycle (Fig. 1) or initiates a radical chain process by reducing a new substrate molecule. Using eosin Y as the photoredox catalyst, Zeitler and coworkers noticed that the reaction proceeded in the dark after an initial irradiation which is an indicator for the involvement of a radical chain process.10 In either case, the catalytic cycle is redox neutral and the reaction catalyzed by the temporary transfer of one electron.5
Table 1 Enantioselective α-alkylation of aldehydes via asymmetric photoredox catalysis

|
REWG-X |
Amines |
PSa |
Conditions |
Light sources |
Yields (%) |
ee (%) |
Ref. |
Abbreviations for ligands: 2,2′-bipyridine (bpy), cyclometalated 2-phenylpyridine (ppy), 4,4′-di-tert-butyl-2,2′-bipyridine (dtbbpy).
One example with CF2BrCO2Et.
Including heteroaryl substrates such as 4-bromomethyl pyridine as its HBr salt.
Diethyl bromomalonate, 4-nitro-phenacyl bromide, and 1-iodononafluorobutane.
Diethyl bromomalonate, phenacyl bromide, 2,4-dinitrobenzyl bromide.
Used as the HCl salt.
64 mg inorganic semiconductor per nmol of bromoorganyl.
Diethyl bromomalonate and phenacyl bromides.
Catalyst loading of ca. 2 mol%.
Bulk powder.
Nanoparticles.
|
α-Bromocarbonyls |
1
|
[Ru(bpy)3]Cl2 |
23 °C, 6 h |
15 W fluorescent lamp |
66–93 |
88–99 |
MacMillan (2008)7 |
Perfluoralkyl iodidesb |
1
|
[Ir(ppy)2(dtbbpy)]PF6 |
−20 °C, 7.5–8 h |
26 W fluorescent lamp |
61–89 |
90–99 |
MacMillan (2009)8 |
Benzyl bromidesc |
2
|
fac-[Ir(ppy)3] |
rt, 3–6 h |
26 W fluorescent lamp |
72–91 |
87–93 |
MacMillan (2010)9 |
Different classesd |
1
|
Eosin Y |
−15 to +5 °C, 15–18 h |
1 W LED, λ = 530 nm |
56–85 |
86–96 |
Zeitler (2011)10 |
Different classese |
1
|
PbBiO2Brg |
20 °C, 20–24 h |
3 W LED, λ = 440 nm |
65–84 |
72–96 |
König (2012)11 |
Different classesh |
1
|
Bi2O3i,j, Bi2S3i,k |
rt, 1–48 h |
23 W fluorescent lamp |
40–91 |
81–98 |
Pericàs (2014)12 |
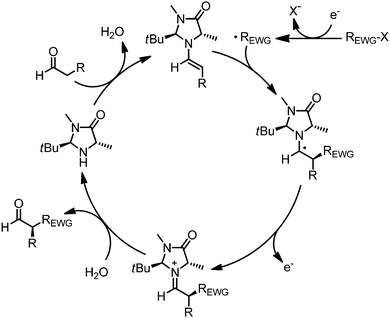 |
| Fig. 2 Putative mechanism for the redox neutral enantioselective α-alkylation of aldehydes via asymmetric photoredox catalysis. | |
Luo and coworkers recently introduced a very useful extension of this asymmetric photoredox enamine catalysis strategy by reporting that the chiral primary amine 3 is a suitable enamine catalyst for the asymmetric photoredox α-alkylation of β-dicarbonyl compounds, thereby implementing all-carbon quaternary stereocenters with high enantioselectivities (Fig. 3).14 The authors propose that a hydrogen bond in the transition state between the protonated tertiary amine (N–H as hydrogen bond donor) of the intermediate enamine and the intermediate phenacyl radical (C
O as hydrogen bond acceptor) contributes to the high asymmetric induction. Interestingly, β-ketoamides bearing N-aryl groups lead to spontaneous intramolecular cyclizations under formation of hemiaminals.
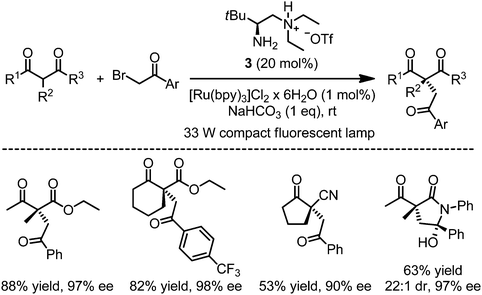 |
| Fig. 3 Asymmetric α-alkylation of β-dicarbonyl compounds via asymmetric photoredox catalysis employing a chiral primary amine as enamine catalyst as reported by Luo. | |
Visible light absorption by transient donor–acceptor complex
Melchiorre and coworkers recently reported a surprising finding, namely that the asymmetric α-alkylation of aldehydes with electron deficient benzyl bromides and phenacyl bromides via photoredox catalysis with the chiral secondary amine 4 can be performed in the absence of any external photoredox sensitizer (Fig. 4a).15 Based on extensive mechanistic investigations, the authors propose a mechanism which proceeds through a transient electron donor acceptor (EDA) complex. This colored charge transfer complex composed of an electron-rich enamine and an electron-deficient acceptor-substituted organobromide is capable of absorbing visible light and triggers a (reversible) single electron transfer from the enamine to the organobromide, thereby producing a solvated contact radical ion pair. Subsequent heterolytic fragmentation of the organobromide radical anion produces bromide and an alkyl radical that combines with the chiral enamine radical cation to generate a non-racemic iminium ion. After hydrolysis, the non-racemic α-alkylated aldehyde is provided. Note that this reaction mechanism is not a classical electron transfer catalyzed reaction since here an electron is not added or removed from the system but instead transferred between an intermediate and a substrate. Regardless, the authors also demonstrate that solar light instead of a fluorescent light bulb can effectively promote this process. The authors expanded this strategy of asymmetric photo-organocatalysis via visible light absorbing charge transfer complexes to the enantioselective α-alkylation of ketones using the cinchona-based primary amine catalyst 5 (Fig. 4b).16
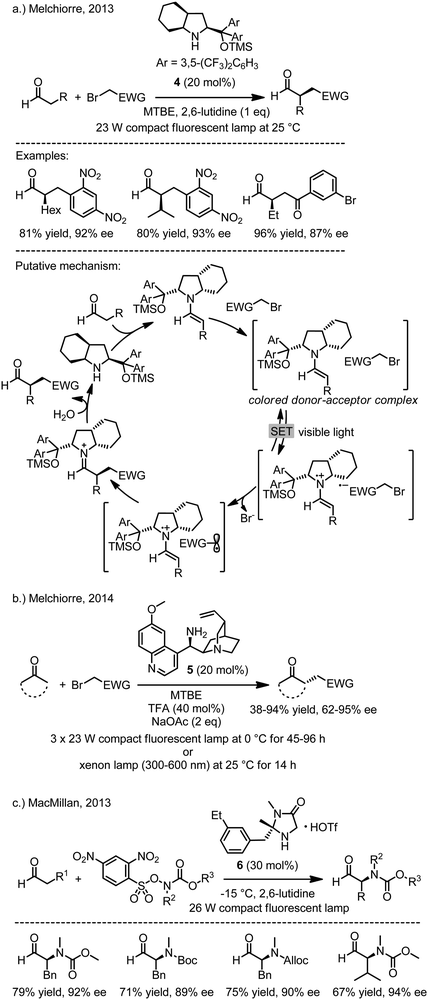 |
| Fig. 4 Enantioselective α-alkylation of aldehydes via asymmetric photo-organocatalysis. Note that these reactions do not require any external photosensitizer. | |
Interestingly, MacMillan and coworkers recently reported a photoinduced enantioselective α-amination of aldehydes using 2,4-dinitrophenylsulfonyloxy-N-functionalized carbamates together with the chiral primary amine 6 (Fig. 4c).17 The reaction apparently proceeds through the formation of an N-centered radicals and their subsequent stereoselective addition to the electron-rich π-bond of chiral enamine intermediates. Importantly, as for Melchiorre’s work, no additional photosensitizer is required.
Asymmetric photoredox catalysis with a chiral iridium complex
Our group recently reported a novel type of asymmetric photoredox catalyst which combines photoredox sensitization, substrate activation, and asymmetric induction in a single chiral iridium complex.18 In the presence of a 14 W energy saving household lamp, 7 at a loading of 2 mol% is able to catalyze the reaction between 2-acyl imidazoles and electron deficient benzyl bromides or phenacyl bromides, providing α-alkylated 2-acyl imidazoles in high yields and with high enantioselectivities. A plausible mechanism in which photoredox catalysis intertwines with asymmetric catalysis is shown in Fig. 5. Herein, the catalysis is initiated by the coordination of 2-acyl imidazoles to the iridium catalyst in a bidentate fashion, followed upon deprotonation by the formation of a nucleophilic iridium(III) enolate complex. The subsequent chirality generating key step constitutes the exergonic addition of a photo-reductively generated electrophilic radical to the electron rich metal-coordinated enolate double bond, thereby affording an iridium-coordinated ketyl radical. Oxidation of this ketyl intermediate to a carbonyl group by single electron transfer provides the iridium-coordinated product, which is subsequently released. The single electron transfer either regenerates the iridium(III) photosensitizer or leads to the reduction of another organobromide substrate, thereby initiating a chain reaction. Proposed key intermediate which uniquely connects the asymmetric catalysis with the photoredox cycle is the highlighted iridium(III) enolate complex, which not only provides the crucial asymmetric induction upon a stereocontrolled reaction with the reductively formed radicals in the catalysis cycle and but at the same time serves as the in situ generated active photosensitizer. Despite the structural simplicity of the metal-based catalyst, the metal center serves multiple functions at the same time: as a precursor for the photosensitizer, as a chiral Lewis acid for substrate activation, and as the exclusive chiral center for providing a highly effective asymmetric induction in the course of the C–C bond forming reaction.
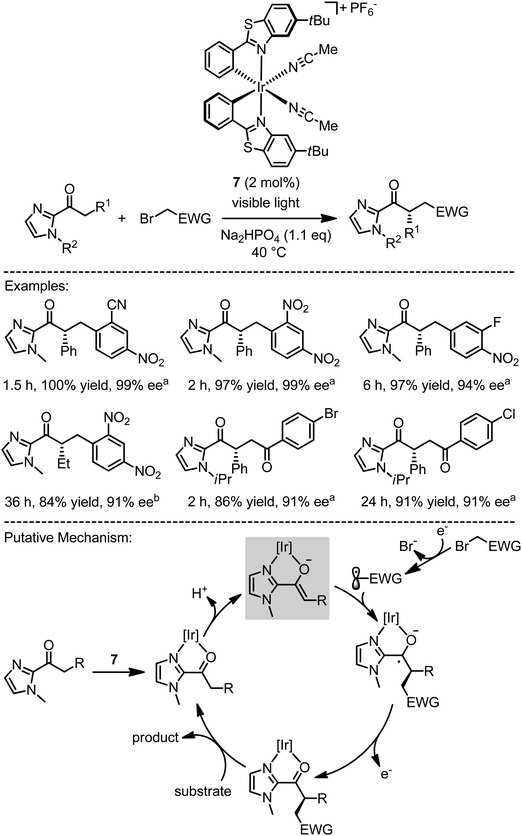 |
| Fig. 5 Plausible mechanism for a combined photoredox and asymmetric catalysis with a chiral iridium Lewis acid photoredox sensitizer. The proposed mechanism involves the highlighted intermediate iridium(III) enolate complex, which most likely serves as the active photosensitizer and the chiral reaction partner for the electron deficient radicals (EWG = electron withdrawing group). a Energy saving household lamp (14 W). b Blue LED light (3 W). | |
Electron transfer catalyzed asymmetric [2+2]-photocycloaddition
[2+2]-Photocycloadditions can be triggered by single electron transfer.19 Yoon recently reported a dual catalyst system consisting of a visible light activated photoredox catalyst and a chiral Lewis acid co-catalyst (Fig. 6).20 From previous work of the authors it was established that [Ru(bpy)3]Cl2, upon activation with visible light and in the presence of an electron donor such as iPr2NEt, is capable of reducing aryl enones that are coordinated to a mild Lewis acid.21 Photoactivated [Ru*(bpy)3]2+ accepts an electron from iPr2NEt to produce the reduced complex [Ru(bpy)3]+ which transfers a single electron to Lewis acid coordinated aryl enones (photoredox sensitizer cycle II in Fig. 1). The hereby generated intermediate radical anions can subsequently participate in efficient [2+2]-cycloaddition reactions with other (less-conjugated) Michael acceptors. The formed cyclobutane containing ketyl radicals loose an electron and thereby close the electron transfer catalysis cycle. The authors demonstrated that in the presence of a chiral Lewis acid, namely Eu(OTf)3 (10 mol%) together with a chiral coordinating ligand (20–30 mol%), the cyclobutane products are formed with high enantio- and modest diastereoselectivities controlled by the chiral Lewis acid which remains coordinated throughout the reaction.20 The authors point out that the high enantioselectivities profit from the fact that no background reaction occurs in the absence of the Lewis acid. Interestingly, modifying the chiral ligand from the Schiff base 8 to the corresponding reduced secondary amine 9 led to a switch in diastereoselectivity from 1,2-trans to 1,2-cis cyclobutanes, thus demonstrating that the chiral ligand can control both the relative and absolute stereochemistry of the [2+2]-photocycloaddition products.
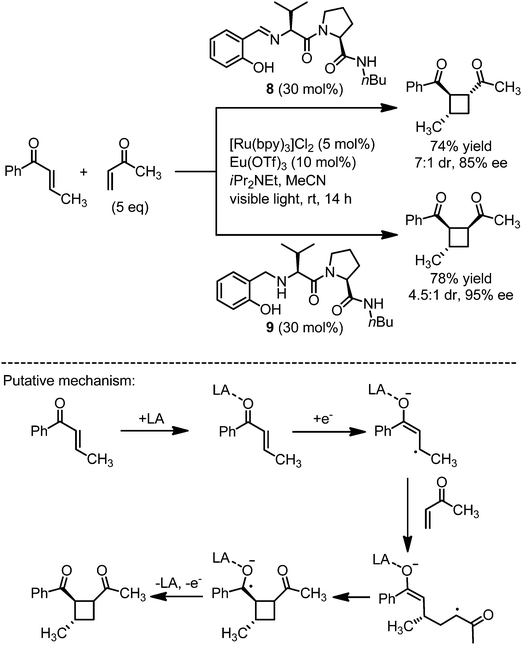 |
| Fig. 6 Dual catalysis system for the enantioselective [2+2]-photocycloaddition of α,β-unsaturated ketones to the corresponding cyclobutanes. LA = chiral Lewis acid catalyst. | |
Photoinduced electron transfer: net reduction or oxidation
Photoredox catalysis combined with chiral Brønsted acid catalysis
Knowles recently reported a very powerful enantioselective reductive aza-pinacol cyclization induced by proton-coupled electron transfer (PCET) (Fig. 7).22,23 Accordingly, the exposure of ε-hydrazino arylketones to blue light in the presence of a photoredox sensitizer (2 mol%), the chiral BINOL phosphoric acid 10 (10 mol%), and a Hantzsch ester as the terminal reducing agent provided the cis-vicinal amino alcohols in an enantioselective fashion. The authors propose a mechanism in which the Brønsted acid forms a hydrogen bond with the aryl ketone, followed by an electron transfer from the photoactivated photosensitizer to the aryl ketone in concert with proton transfer from the Brønsted acid (pKa = 13 in MeCN) to the oxygen of the formed ketyl (pKa = 20 in MeCN). What makes the concept of PCET so attractive is the fact that it allows to reduce functional groups at significant lower oxidation potentials and provides an opportunity to achieve asymmetric induction by using chiral Brønsted acids. The enantioselective radical cyclization of the ketyl radical can be rationalized if the chiral phosphate remains hydrogen bonded to the OH-group of the ketyl, which is quite surprising considering that the association apparently occurs mainly through a single H-bond. Subsequently, the product is formed after hydrogen atom abstraction of the intermediate amino radical from Hantzsch ester which is then further oxidized to its corresponding pyridinium ion upon regeneration of the photosensitizer.
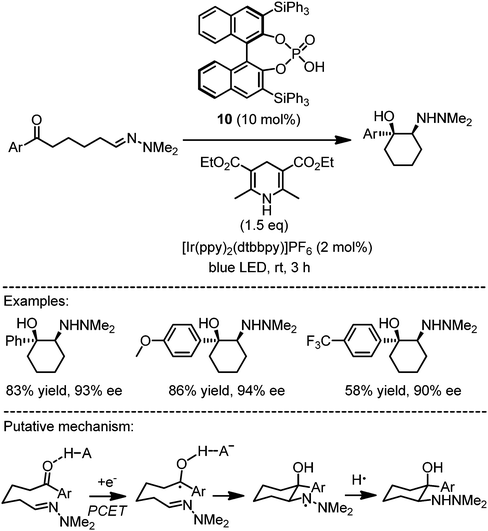 |
| Fig. 7 Asymmetric aza-pinacol cyclization by merged photoredox catalysis and chiral Brønsted acid catalysis. | |
Tandem iminium/enamine photoredox catalysis
An oxidative strategy to exploit the reactivity of enamine intermediates has been termed SOMO catalysis and makes use of the low ionization potential of enamines.24 Jang and coworkers recently reported a strategy to combine asymmetric SOMO catalysis with photoredox catalysis (Fig. 8).25 Accordingly, the reaction of α,β-unsaturated aldehydes with dialkyl malonates and the radical scavenger 2,2,6,6-tetramethylpiperidine-1-oxyl (TEMPO) in the presence of the proline derivative 11 and a visible light activated photosensitizer provided in a cascade Michael addition/oxyamination reaction sequence non-racemic aldehydes with stereocenters at both the α- and β-positions. As the photoredox sensitizer served a TiO2-bound ruthenium(II) complex (N719) that has been developed previously for Grätzel-type dye-sensitized solar cells. Mechanistically, the reaction apparently combines iminium activation with SOMO activation as shown in Fig. 8.
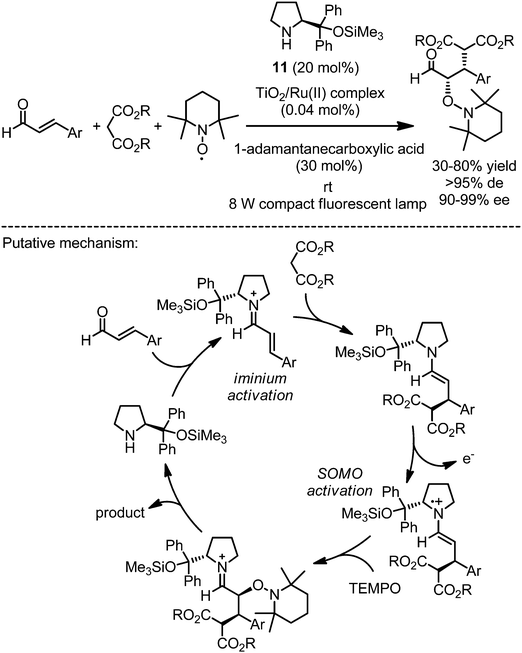 |
| Fig. 8 Asymmetric tandem Michael addition/oxyamination under photoredox conditions. | |
Photoredox catalysis combined with N-heterocyclic carbene catalysis
Amines can be converted oxidatively to iminium ions. If the oxidation is mediated by a photoredox sensitizer, this process contains an initial single electron transfer step to an acidic amine radical cation, which after α-deprotonation to an α-aminoradical is prone to a further single electron oxidation under formation of an electrophilic iminium ion.2–4 DiRocco and Rovis exploited this for an enantioselective photosensitized oxidative coupling of tetrahydroisoquinolines with aldehydes, catalyzed by the chiral N-heterocyclic carbene (NHC) catalyst 12 (Fig. 9).26 Apparently, the reaction of aldehydes with the NHC catalyst affords a nucleophilic chiral Breslow intermediate which is trapped by an electrophilic iminium ion, thereby providing an enantioselective α-acylation of N-aryltetrahydroisoquinolines via dual catalysis by an N-heterocyclic carbene and a photoredox sensitizer. Importantly, the presence of the weak oxidant m-dinitrobenzene turns out to be essential for achieving a high catalytic efficiency in this process and it is likely that m-dinitrobenzene serves as a redox mediator between the photoexcited [Ru*(bpy)3]2+ and oxygen as the terminal oxidant.
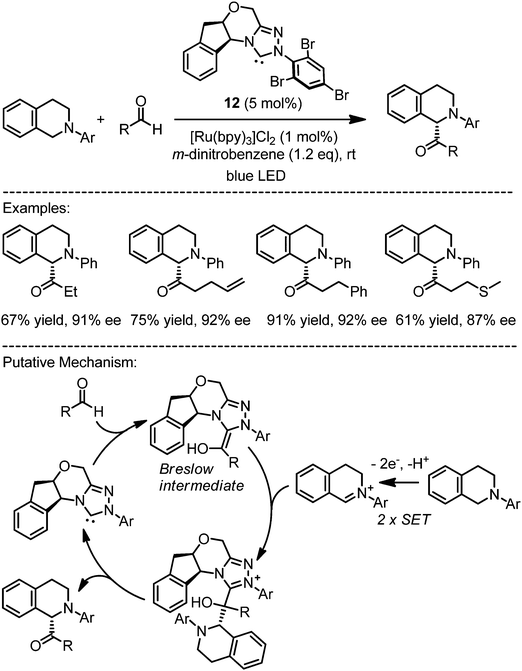 |
| Fig. 9 Asymmetric α-acylation of N-aryltetrahydroisoquinolines via dual catalysis by an N-heterocyclic carbene and a photoredox sensitizer. | |
Photoredox catalysis combined with asymmetric hydrogen bonding catalysis
Jacobsen and Stephenson accomplished the enantioselective alkylation of N-aryltetrahydroisoquinolines by using a chiral thiourea hydrogen bonding catalyst in a sequential two-step procedure (Fig. 10).27 Accordingly, in the first step, a photoredox mediated oxidation with CCl4 as the final oxidant leads to α-chloroamines. In a second step, upon change to a less polar solvent and a reduction of the temperature, the addition of the chiral thiourea 13 presumably results in the formation of an intermediate contact ion pair out of a hydrogen-bonded chloride anion and the iminium cation, which reacts enantioselectively with the silyl enol ether to provide the products with modest overall yields but high enantioselectivities.
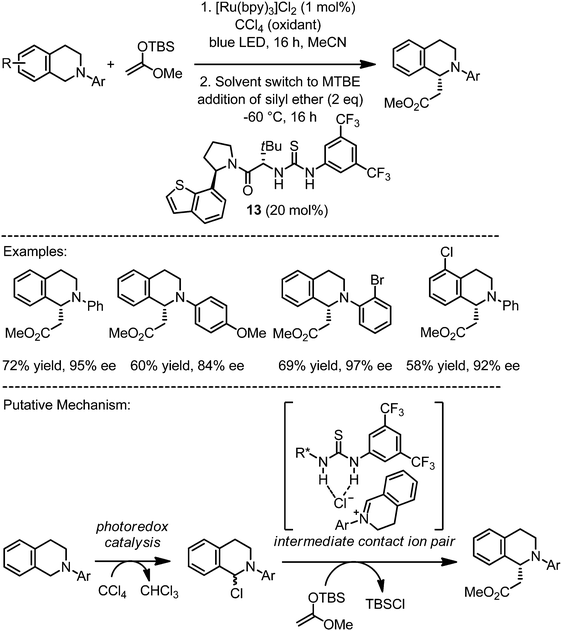 |
| Fig. 10 Asymmetric α-alkylation of N-aryltetrahydroisoquinolines via sequential catalysis through photosensitized oxidation followed by asymmetric anion binding catalysis with a chiral thiourea. | |
Photoinduced energy transfer processes
Asymmetric reactions with singlet oxygen
Molecular oxygen is considered a green oxidant as it is highly abundant and typically reduced to just water. Whereas molecular oxygen, due to its biradical character, readily reacts with other radicals often even in a diffusion controlled fashion, the reactivity towards closed shell molecules is low. However this reactivity can be increased dramatically by converting triplet oxygen (3O2) to singlet oxygen (1O2) through energy transfer from the singlet state of an excited photosensitizer (Fig. 1).28 The reactivity of singlet oxygen was applied to visible light activated asymmetric catalysis in a few recent studies.29–33 Córdova developed an enantioselective α-oxidation of aldehydes and ketones using molecular oxygen as shown in Fig. 11.29–31 For example, the reaction of aldehydes with molecular oxygen in the presence of the proline derivative 11 (20 mol%) and meso-tetraphenylporphyrin (TPP, 1 mol%) at 0 °C for 6 hours, afforded non-racemic 1,2-diols after in situ reduction with NaBH4.31 The key step in the proposed mechanism is the enantioselective reaction between an intermediate nucleophilic chiral enamine and singlet oxygen, albeit with just a modest asymmetric induction, presumably as a consequence of the small size of molecular oxygen. In a similar fashion, the amino acid alanine (20 mol%) was reported to catalyze the analogous α-oxidation of ketones, whereas the cyclic amino acid proline afforded inferior enantioselectivities.30 These α-oxygenations are inexpensive and environmentally benign since catalyst and oxidant are from renewable sources.
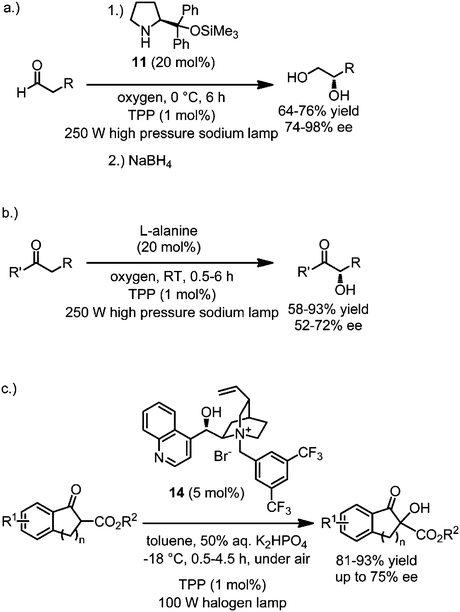 |
| Fig. 11 Direct α-oxidation of aldehydes, ketones, and β-keto esters with molecular oxygen catalyzed by a chiral secondary amine or a chiral phase transfer catalyst in combination with a the photosensitizer tetraphenylporphyrin (TPP). | |
Gao and coworkers used a somewhat different strategy to control the enantioselectivity in α-hydroxylations with molecular oxygen by employing a chiral phase transfer catalyst.32 When treated with the chiral phase catalyst 14 (5 mol%) and the photosensitizer TPP (1 mol%) under basic phase transfer conditions while exposing to visible light under an atmosphere of air, the α-hydroxylated products were obtained in high yields in an enantioselective fashion. No products were obtained upon addition of the 1O2 scavenger 1,4-diazabicyclo[2.2.2]octane. The authors propose that the reaction proceeds through a contact ion pair out of the quaternary ammonium ion of the chiral catalyst 14 and the α-deprotonated β-ketoester, which then reacts with the electrophile singlet oxygen in a stereocontrolled fashion to form an intermediate hydroperoxide which itself serves as an oxidant for another deprotonated β-ketoester to form the final hydroxylated product in an enantioselective fashion.
Asymmetric visible light [2+2]-photocycloaddition
The Bach group recently reported an organocatalyst (15) for enantioselective intramolecular [2+2]-photocycloaddition reactions induced by visible light (Fig. 12).34,35 The catalyst is based on a 1,5,7-trimethyl-3-azabicyclo[3.3.1]nonan-2-one scaffold connected to a thioxanthone chromophore via an oxazole moiety and catalyzes the conversion of 4-substituted chinolones to their intramolecular cycloaddition products activated by visible light. The mechanistic model assumes the binding of the 4-substituted chinolones to the catalyst through a double hydrogen bond as depicted in Fig. 12. In contrast to related chiral photosensitizers requiring activation by UV-light,36 thioxanthones absorb visible light (λmax = 387 nm). The thioxanthone serves as the light-harvesting antenna and transfers the energy of the absorbed photon to the chinolone, presumably by triplet-energy transfer. Importantly, the planar thioxanthone moiety not only constitutes the chromophore but also provides the enantiodifferentiation by allowing the attack of the quinolone double bond only from one prochiral face, thereby affording the cycloaddition product in an enantioselective fashion. The authors mention that the catalyst can be recovered in high yields after the reaction, demonstrating that light-induced catalyst decomposition is not severe. This work belongs to the few examples in which a single catalyst is capable to catalyze an asymmetric transformation activated by visible light.
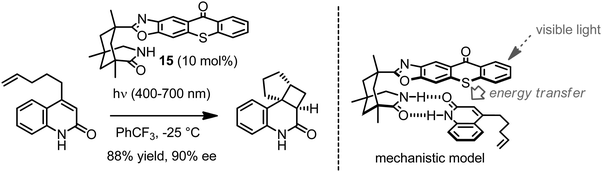 |
| Fig. 12 Visible light induced intramolecular enantioselective [2+2]-photocycloaddition catalyzed by a chiral thioxanthone. An example reaction with 4-(pent-4-enyl)quinolone is shown on the left and a mechanistic model on the right. | |
Conclusions
Despite exciting recent progress, photoactivated asymmetric catalysis is an area of research that is still in its infancies and builds on pioneering contributions by Bach (UV light activated asymmetric catalysis6,36) and MacMillan (visible light activated asymmetric catalysis7–9). The current large interest in exploiting visible light as an abundant source of energy for activating chemical reactions is not only due to a prevailing environmental consciousness, but rather also attributed to the fact that photochemistry allows to generate highly reactive intermediates under mild reaction conditions. Thereby formed intermediate radical ions and radicals are themselves highly reactive and it is therefore often challenging to control and direct their reactivity with respect to functional group selectivity and stereoselectivity. However, the near future will certainly witness new strategies for interfacing the advantages of visible light activated photochemistry with asymmetric catalysis.37,38 For this, future work needs to identify new types of catalytic asymmetric processes that are especially suitable for an activation by visible light induced electron or energy transfer, and this may include the design of fine tuned and chiral photosensitizers.39
Acknowledgements
I would particularly like to thank my coworkers Haohua Huo, Xiaodong Shen and Chuanyong Wang for their pioneering contributions to this new area of research in our laboratories. This project was funded in parts by the German Research Foundation (ME1805/4-1 and ME1805/11-1).
References
- H.-J. Federsel, Nat. Rev. Drug Discovery, 2005, 4, 685–697 CrossRef CAS PubMed.
- For reviews on visible light photocatalysis, see:
(a) K. Zeitler, Angew. Chem., Int. Ed., 2009, 48, 9785–9789 CrossRef CAS PubMed;
(b) T. P. Yoon, M. A. Ischay and J. Du, Nat. Chem., 2010, 2, 527–532 CrossRef CAS PubMed;
(c) J. M. R. Narayanam and C. R. J. Stephenson, Chem. Soc. Rev., 2010, 40, 102–113 RSC;
(d) F. Teplý, Collect. Czech. Chem. Commun., 2011, 76, 859–917 CrossRef;
(e) J. W. Tucker and C. R. J. Stephenson, J. Org. Chem., 2012, 77, 1617–1622 CrossRef CAS PubMed;
(f) J. Xuan and W.-J. Xiao, Angew. Chem., Int. Ed., 2012, 51, 6828–6838 CrossRef CAS PubMed;
(g) D. P. Hari and B. König, Angew. Chem., Int. Ed., 2013, 52, 4734–4743 CrossRef CAS PubMed;
(h) C. K. Prier, D. A. Rankic and D. W. C. MacMillan, Chem. Rev., 2013, 113, 5322–5363 CrossRef CAS PubMed;
(i) D. M. Schultz and T. P. Yoon, Science, 2014, 343, 1239176 CrossRef PubMed.
-
(a) J. Mattay, Angew. Chem., Int. Ed. Engl., 1987, 26, 825–845 CrossRef;
(b) M. Fagnoni, D. Dondi, D. Ravelli and A. Albini, Chem. Rev., 2007, 107, 2725–2756 CrossRef CAS PubMed.
- For the reactivity of radical ions, see:
(a) P. S. Mariano, Acc. Chem. Res., 1983, 16, 130–137 CrossRef CAS;
(b) N. L. Bauld, Tetrahedron, 1989, 45, 5307–5363 CrossRef CAS;
(c) U. C. Yoon and P. S. Mariano, Acc. Chem. Res., 1992, 25, 233–240 CrossRef CAS;
(d) G. Pandey, Synlett, 1992, 546–552 CrossRef CAS PubMed;
(e) M. Schmittel and A. Burghart, Angew. Chem., Int. Ed. Engl., 1997, 36, 2550–2589 CrossRef;
(f) J.-i. Yoshida and K. Nishiwaki, J. Chem. Soc., Dalton Trans., 1998, 2589–2596 RSC;
(g) N. J. Saettel, J. Oxgaard and O. Wiest, Eur. J. Org. Chem., 2001, 1429–1439 CrossRef CAS;
(h) P. J. Donoghue and O. Wiest, Chem. – Eur. J., 2006, 12, 7018–7026 CrossRef CAS PubMed;
(i) K. Moeller, Synlett, 2009, 1208–1218 CrossRef CAS PubMed;
(j) M. A. Ischay and T. P. Yoon, Eur. J. Org. Chem., 2012, 3359–3372 CrossRef CAS;
(k) J. Hu, J. Wang, T. H. Nguyen and N. Zheng, Beilstein J. Org. Chem., 2013, 9, 1977–2001 CrossRef PubMed;
(l) M. Reckenthäler and A. G. Griesbeck, Adv. Synth. Catal., 2013, 355, 2727–2744 CrossRef;
(m) N. Zhang, S. R. Samanta, B. M. Rosen and V. Percec, Chem. Rev., 2014, 114, 5848–5958 CrossRef CAS PubMed.
- A. Studer and D. P. Curran, Nat. Chem., 2014, 6, 765–773 CrossRef CAS PubMed.
- For an early example of catalytic enantioselective chemistry driven by UV light induced electron transfer, see: A. Bauer, F. Westkämper, S. Grimme and T. Bach, Nature, 2005, 436, 1139–1140 CrossRef CAS PubMed.
- D. A. Nicewicz and D. W. C. MacMillan, Science, 2008, 322, 77–80 CrossRef CAS PubMed.
- D. A. Nagib, M. E. Scott and D. W. C. MacMillan, J. Am. Chem. Soc., 2009, 131, 10875–10877 CrossRef CAS PubMed.
- H.-W. Shih, M. N. Vander Wal, R. L. Grange and D. W. C. MacMillan, J. Am. Chem. Soc., 2010, 132, 13600–13603 CrossRef CAS PubMed.
- M. Neumann, S. Füldner, B. König and K. Zeitler, Angew. Chem., Int. Ed., 2011, 50, 951–954 CrossRef CAS PubMed.
- M. Cherevatskaya, M. Neumann, S. Füldner, C. Harlander, S. Kümmel, S. Dankesreiter, A. Pfitzner, K. Zeitler and B. König, Angew. Chem., Int. Ed., 2012, 51, 4062–4066 CrossRef CAS PubMed.
- P. Riente, A. MatasAdams, J. Albero, E. Palomares and M. A. Pericàs, Angew. Chem., Int. Ed., 2014, 53, 9613–9616 CrossRef CAS PubMed.
-
D. P. Curran, N. A. Porter and B. Giese, Stereochemistry of Radical Reactions, VCH, Weinheim, 1996 Search PubMed.
- Y. Zhu, L. Zhang and S. Luo, J. Am. Chem. Soc., 2014, 136, 14642–14645 CrossRef CAS PubMed.
- E. Arceo, I. D. Jurberg, A. Álvarez-Fernández and P. Melchiorre, Nat. Chem., 2013, 5, 750–756 CrossRef CAS PubMed.
- E. Arceo, A. Bahamonde, G. Bergonzini and P. Melchiorre, Chem. Sci., 2014, 5, 2438–2442 RSC.
- G. Cecere, C. M. König, J. L. Alleva and D. W. C. MacMillan, J. Am. Chem. Soc., 2013, 135, 11521–11524 CrossRef CAS PubMed.
- H. Huo, X. Shen, C. Wang, L. Zhang, P. Röse, L.-A. Chen, K. Harms, M. Marsch, G. Hilt and E. Meggers, Nature, 2014, 515, 100–103 CrossRef CAS PubMed.
- For chemically and electrochemically induced radical anion [2+2]-cycloadditions of bis(enones), see:
(a) Y. Roh, H.-Y. Jang, V. Lynch, N. L. Bauld and M. J. Krische, Org. Lett., 2002, 4, 611–613 CrossRef CAS PubMed;
(b) J. Yang, G. A. N. Felton, N. L. Bauld and M. J. Krische, J. Am. Chem. Soc., 2004, 126, 1634–1635 CrossRef CAS PubMed.
- J. Du, K. L. Skubi, D. M. Schultz and T. P. Yoon, Science, 2014, 344, 392–396 CrossRef CAS PubMed.
-
(a) M. A. Ischay, M. E. Anzovino, J. Du and T. P. Yoon, J. Am. Chem. Soc., 2008, 130, 12886–12887 CrossRef CAS PubMed;
(b) J. Du and T. P. Yoon, J. Am. Chem. Soc., 2009, 131, 14604–14605 CrossRef CAS PubMed.
- L. J. Rono, H. G. Yayla, D. Y. Wang, M. F. Armstrong and R. R. Knowles, J. Am. Chem. Soc., 2013, 135, 17735–17738 CrossRef CAS PubMed.
- H. G. Yayla and R. R. Knowles, Synlett, 2014, 2819–2826 CAS.
- T. D. Beeson, A. Mastracchio, J.-B. Hong, K. Ashton and D. W. C. MacMillan, Science, 2007, 316, 582–585 CrossRef CAS PubMed.
- H.-S. Yoon, X.-H. Ho, J. Jang, H.-J. Lee, S.-J. Kim and H.-Y. Jang, Org. Lett., 2012, 14, 3272–3275 CrossRef CAS PubMed.
- D. A. DiRocco and T. Rovis, J. Am. Chem. Soc., 2012, 134, 8094–8097 CrossRef CAS PubMed.
- G. Bergonzini, C. S. Schindler, C.-J. Wallentin, E. N. Jacobsen and C. R. J. Stephenson, Chem. Sci., 2013, 5, 112–116 RSC.
- E. L. Clennan and A. Pace, Tetrahedron, 2005, 61, 6665–6691 CrossRef CAS PubMed.
- A. Córdova, H. Sundén, M. Engqvist, I. Ibrahem and J. Casas, J. Am. Chem. Soc., 2004, 126, 8914–8915 CrossRef PubMed.
- H. Sundén, M. Engqvist, J. Casas, I. Ibrahem and A. Córdova, Angew. Chem., Int. Ed., 2004, 43, 6532–6535 CrossRef PubMed.
- I. Ibrahem, G.-L. Zhao, H. Sundén and A. Córdova, Tetrahedron Lett., 2006, 47, 4659–4663 CrossRef CAS PubMed.
- M. Lian, Z. Li, Y. Cai, Q. Meng and Z. Gao, Chem. – Asian J., 2012, 7, 2019–2023 CrossRef CAS PubMed.
- For an interesting template-mediated enantioselective, visible light activated [2+4]-cycloaddition with singlet oxygen, see: C. Wiegand, E. Herdtweck and T. Bach, Chem. Commun., 2012, 48, 10195–10197 RSC.
- R. Alonso and T. Bach, Angew. Chem., Int. Ed., 2014, 53, 4368–4371 CrossRef CAS PubMed.
- For a very recent example of an enantioselective [2+2]-photocycloaddition catalyzed by chiral thiourea in the presence of UV-light, see: N. Vallavoju, S. Selvakumar, S. Jockusch, M. P. Sibi and J. Sivaguru, Angew. Chem., Int. Ed., 2014, 53, 5604–5608 CrossRef CAS PubMed.
-
(a) C. Müller, A. Bauer and T. Bach, Angew. Chem., Int. Ed., 2009, 48, 6640–6642 CrossRef PubMed;
(b) H. Guo, E. Herdtweck and T. Bach, Angew. Chem., Int. Ed., 2010, 49, 7782–7785 CrossRef CAS PubMed;
(c) C. Müller, A. Bauer, M. M. Maturi, M. C. Cuquerella, M. A. Miranda and T. Bach, J. Am. Chem. Soc., 2011, 133, 16689–16697 CrossRef PubMed;
(d) S. C. Coote and T. Bach, J. Am. Chem. Soc., 2013, 135, 14948–14951 CrossRef CAS PubMed;
(e) R. Brimioulle and T. Bach, Science, 2013, 342, 840–843 CrossRef CAS PubMed.
- Mechanistically not yet well understood is interesting work reported by Katsuki and coworkers regarding visible light activated asymmetric oxidative chemistry with chiral Ru(NO) salen complexes. See:
(a) R. Irie, K. Masutani and T. Katsuki, Synlett, 2000, 1433–1436 CAS;
(b) H. Shimizu, S. Onitsuka, H. Egami and T. Katsuki, J. Am. Chem. Soc., 2005, 127, 5396–5413 CrossRef CAS PubMed;
(c) H. Tanaka, H. Nishikawa, T. Uchida and T. Katsuki, J. Am. Chem. Soc., 2010, 132, 12034–12041 CrossRef CAS PubMed.
- A few interesting catalytic asymmetric transformations catalyzed by visible light, but which provide only low to modest enantioselectivities, have not been discussed here. See:
(a) T. Hamada, H. Ishida, S. Usui, Y. Watanabe, K. Tsumura and K. Ohkubo, J. Chem. Soc., Chem. Commun., 1993, 909–911 RSC;
(b) M. T. Pirnot, D. A. Rankic, D. B. C. Martin and D. W. C. MacMillan, Science, 2013, 339, 1593–1596 CrossRef CAS PubMed;
(c) J. C. Tellis, D. N. Primer and G. A. Molander, Science, 2014, 345, 433–436 CrossRef CAS PubMed;
(d) Z.-J. Feng, J. Xuan, X.-D. Xia, W. Ding, W. Guo, J.-R. Chen, Y.-Q. Zou, L.-Q. Lu and W.-J. Xiao, Org. Biomol. Chem., 2014, 12, 2037–2040 RSC;
(e) W. Ding, Q.-Q. Zhou, J. Xuan, T.-R. Li, L.-Q. Lu and W.-J. Xiao, Tetrahedron Lett., 2014, 55, 4648–4652 CrossRef CAS PubMed.
- Photosensitizer deactivation pathways should also be taken into account. See: J. J. Devery III, J. J. Douglas, J. D. Nguyen, K. P. Cole, R. A. Flowers II and C. R. J. Stephenson, Chem. Sci., 2015, 6, 537–541 RSC.
|
This journal is © The Royal Society of Chemistry 2015 |