DOI:
10.1039/C5SC03251B
(Edge Article)
Chem. Sci., 2015,
6, 6731-6738
Oxatub[4]arene: a smart macrocyclic receptor with multiple interconvertible cavities†
Received
31st August 2015
, Accepted 11th September 2015
First published on 14th September 2015
Abstract
There are a large number of synthetic macrocyclic receptors in the literature. No particular one is suitable for all guests or purposes. For a broader guest binding scope or multiple purposes, a macrocycle with multiple interconvertible cavities will be advantageous. Here, we report a naphthalene-based macrocyclic receptor with an adaptable cavity, namely oxatub[4]arene. It has four representative conformations resulting from the flipping of naphthalene rings, each with a deep and well-defined cavity. Different guests select one host conformer or a combination of conformers. All the four conformers have been detected and characterized based on 2D NMR spectra and X-ray single crystal structures. Thermodynamically, these conformers constitute a reservoir, that responds to the structural changes of guests, and thus maximizes the association free energies. This smart macrocycle may provide a new platform for the construction of molecular machines and devices or stimuli-responsive materials.
Introduction
Macrocyclic receptors1 are the major workhorses in supramolecular chemistry. These compounds not only contribute to the basic understanding on molecular recognition,2 but also find applications in molecular machines and devices,3 supramolecular polymers,4 stimuli-responsive materials,5 and drug-delivery systems.6 During the last decade, a number of new macrocyclic receptors with novel properties have been reported, including heterocalix[n]aromatics,7 pillar[n]arene,8 bambus[n]uril,9 “Texas-sized” box,10 cyanostar,11 molecular triangles,12 ExBox and ExCage,13 calix[n]imidazole,14 biphen[n]arene,15 coron[n]arene,16 and others.17 These macrocycles show very different guest binding properties, and have certainly enriched the toolbox of supramolecular chemists.
For a given guest or purpose, one of these macrocycles will be the best. Minor structural change of guests or a different kind of guests may significantly affect the corresponding binding affinity. No one macrocycle is suitable for all guests or purposes. Can we design a macrocyclic receptor at least for a broader guest binding scope or multiple purposes? One possibility is to connect several macrocycles covalently together. Thus, the resulting receptor will have all the abilities of these macrocycles. However, its synthesis is too tedious, and when binding a specific guest, only one of these macrocycles in the receptor is used and others are idle. A more ideal receptor should possess several interconvertible cavities and can choose an appropriate one for a given guest to obtain an optimal binding. Recently, we employed dynamic combinatorial chemistry18 and constructed a pair of dynamic configurational macrocycles which can interconvert.19 The two configurations have different cavity parameters and different guests can thermodynamically select different host isomers. Research on similar but covalent receptors confirms the two isomers have very different guest preferences and binding abilities.20 This dynamic system is already close to the “ideal” macrocycle with multiple interconvertible cavities, but it is still a complex mixture.
How can we unify several interconvertible cavities into one kinetically inert macrocycle? Conformational change may be resorted to. Calix[n]arene21 and related compounds are well known to possess several conformations, but not all the conformers have a well-defined cavity. Even though they can interconvert, not all of them show satisfactory guest binding properties. To the best of our knowledge, this kind of smart macrocycles have not been well demonstrated. In the present research, we report a naphthalene-based macrocyclic receptor with four interconvertible and deep cavities. This macrocycle can choose an appropriate cavity for a specific guest and achieve the optimal binding.
Results and discussion
Design and synthesis of oxatub[4]arene
Naphthalene-based macrocycles are relatively rare in the supramolecular literature.19a,20,22,23,24,25 The large π system is expected to create a macrocycle with a deep or wide cavity; however, most of known naphthalene-based macrocycles often suffer from complicated regioisomeric products with ill-defined and shallow cavities, and rarely show satisfactory guest binding performance.
In the present research, we designed and synthesized a naphthalene-based macrocycle (Fig. 1a) and gave it a trivial name – oxatub[4]arene in view of its tube-like cavities and the O atom in the linker (in analogy to oxacalix[3]arene26). Oxatub[4]arene has the following features in its structure: (a) a longer linker is used instead of the often-seen methylene linker. The short linker is believed to cause steric hindrance between neighboring naphthalenes, and the macrocycles may twist, resulting in ill-defined cavities. A longer linker may solve this problem, leading to a macrocycle with a deep cavity. (b) Specifically, the linker CH2–O–CH2, as used in oxacalix[3]arene,26 is employed. Thus, macrocyclization might be readily achieved through Williamson ether synthesis. In addition, the linker may not be innocent and may get involved in binding when the ether oxygen atoms are inwardly-directed. (c) The use of 2,6-dihydroxynaphthalene as the repeating unit and the linking on its 1,5-positions minimize potential isomerism, and also avoid the self-occupation of the cavity as observed in zorb[4]arene,24c in favour of maintaining deep cavities and good host–guest binding. (d) More importantly, the flipping of naphthalene rings leads to multiple conformers with deep cavities.
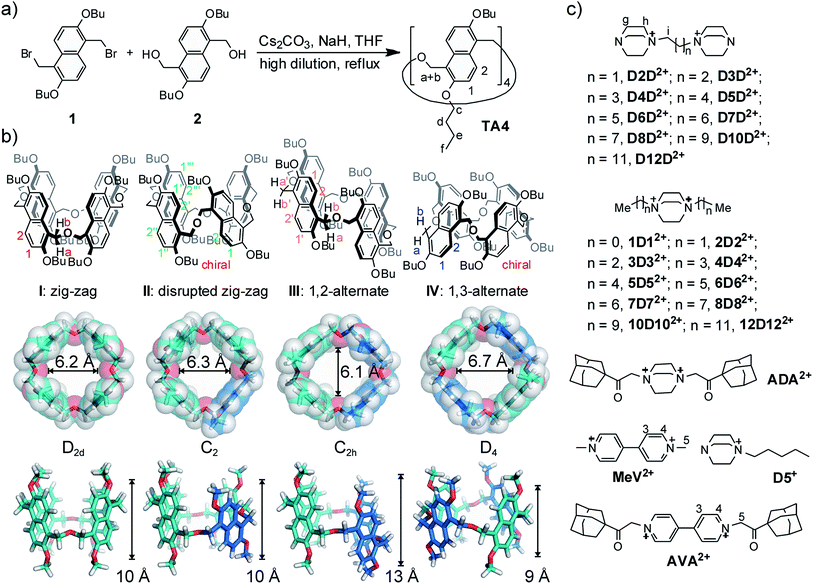 |
| Fig. 1 (a) The synthetic procedure of octabutyl oxatub[4]arene (TA4); (b) chemical structures of four representative conformers of TA4 resulting from naphthalene flipping, and the top and side views of their models. In order to show the cavity in the most expanded forms, the models were obtained by forcing all the methylene protons in the linkers directed straight outwards and the oxygen inwards. Numbering on the structures corresponds to the assignment of NMR signals. Conformers II and IV are chiral, but only one enantiomer is shown here. The lengths of the cavities are based on the distance between two oxygen atoms on the same naphthalene ring. The flipped naphthalene rings in conformations II, III, and IV relative to conformation I are colored in blue. Butyl groups in the models were abbreviated to methyl groups for viewing clarity; (c) chemical structures of all the guests involved in this research; the counterions are PF6−. | |
The synthesis of oxatub[4]arene is straightforward (Fig. 1a). Both the precursors 1 and 2 can be obtained in three steps from commercial materials with or without simple column chromatography. The octabutyl oxatub[4]arene (TA4) was synthesized with a reasonable yield (21%) in a one-pot reaction under a high-dilution condition. The hexamer oxatub[6]arene was also detected by ESI-MS, but remains elusive to isolation.
Properties of oxatub[4]arene
TA4 is a flexible macrocycle and thus possesses numerous conformations. However, the flipping of naphthalene rings as the major activation barriers separates four representative conformations or conformational sets (Fig. 1b). Analogous to calix[4]arene, we name these four conformers as zigzag (I), disrupted zigzag (II), 1,2-alternate (III), and 1,3-alternate (IV), respectively. In order to show the cavity's size and dimension, the models of these conformers are presented in the most expanded form by forcing all the methylene protons in the linkers directed outwards and the oxygen atoms inwards. In contrast to calix[4]arenes, all four conformers possess well-defined cavities which may offer good guest binding properties. Moreover, the cavity of each conformer is different. The diameter and length of conformer I (D2d) are 6.2 and 10 Å, respectively. This is similar to conformer II which, however, has the lowest symmetry (C2). Conformer III (C2h) has a much deeper cavity than the other conformers, while conformer IV (D4) has a much wider cavity. The differences in cavity sizes and lengths are expected to result in different guest binding preferences. According to their different symmetries, 1H NMR can be used to distinguish them when the exchange of guests is slow on the NMR timescale.
The 1H NMR spectrum of TA4 is surprisingly simple and clean (Fig. 2a). It seems there is only one conformer (I or IV according to their symmetries). However, the methylene protons on CH2–O–CH2 appear as a singlet, which should be two doublets (diastereotopic protons) for either conformer I or IV. Only one explanation can account for this: the two protons on the same methylene group may exchange their positions very quickly and this can only occur through a rapid flipping of the naphthalene rings. In order to slow down the ring flipping, variable-temperature NMR experiments (Fig. S1†) were performed. At temperatures below −60 °C, all the peaks are significantly broadened and new peaks appear. One may argue this is caused by aggregation at low temperatures. However, NMR experiments at −60 °C but different concentrations (Fig. S2†) rules out this possibility. At −80 °C, a very complex NMR spectrum was obtained, suggesting the existence of several conformers of TA4. Consequently, there is a conformational ensemble of TA4 in solution. These conformers undergo quick interconversion at room temperature.
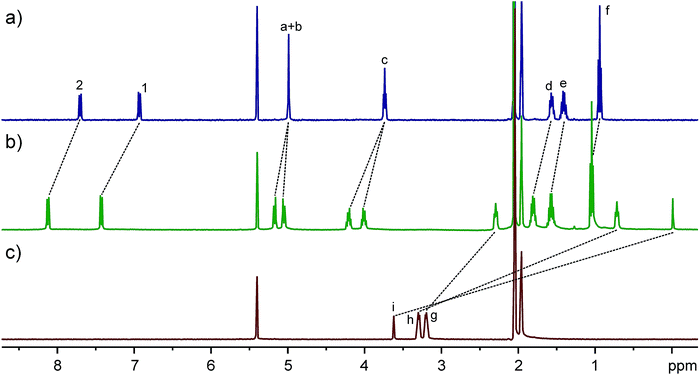 |
| Fig. 2 Full 1H NMR spectra (400 MHz, CD2Cl2 : CD3CN = 1 : 1, 2.0 mM, 25 °C) of (a) TA4, (c) D2D2+, and (b) their equimolar mixture. See Fig. 1 for signal assignments. | |
Host–guest properties and assignments of conformations
Computations indicated all the conformers are electron-rich in their cavities (Fig. S3†), suggesting organic cations would be good guests. The host–guest chemistry of TA4 was initially tested with guest D2D2+. The 1H NMR spectrum (Fig. 2) shows that the signals of both host and guest undergo very large shifts, indicating a binding event. Control experiments (Fig. S4†) with excess guest or host support a slow exchange on the NMR timescale. Free guest or host is not detected, providing evidence for a very strong binding (Ka > 104 M−1). The NMR integrals and mass spectrum (Fig. S6†) suggest a 1
:
1 binding stoichiometry. Meanwhile, the flipping of the naphthalene rings is slow, since the cavity is occupied and the guest exchange is slow. This was further supported by the splitting of proton signals a, b and c (diastereotopic protons). The aromatic protons of the hosts appear as two doublets. According to the conformers' symmetries, the host in this solution can be assigned to a single conformer: either I (D2d) or IV (D4). That is, only one conformer was selected from the conformational ensemble. The other three conformers are not detected at all, suggesting the binding constant of D2D2+ to the conformer present to be at least one order of magnitude larger than those to the other conformers.
Encouraged by the initial results, we systematically studied guests D2D2+–D12D2+ (Fig. 3a and S5†). For guest D3D2+, two new aromatic peaks appeared, indicating the existence of a new host conformer in addition to the conformer observed for D2D2+. These two peaks become more prominent in the case of D4D2+, and dominate for D5D2+. The peaks are slightly broadened. In the case of D6D2+, proton peaks a + b split into two signals, suggesting the ring flipping and guest exchange is still slow at the NMR timescale. This conclusion is supported by use of the similar but monotopic guest D5+. Accordingly, the new conformer can be assigned to either conformer I or IV. That is, both conformers I and IV coexist in the cases of D3D2+ and D4D2+.
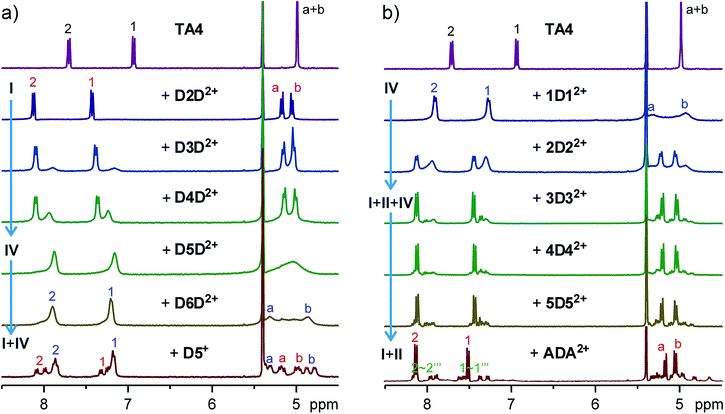 |
| Fig. 3 Partial 1H NMR spectra (400 MHz, CD2Cl2 : CD3CN = 1 : 1, 2.0 mM, 25 °C) of TA4 in the presence of one equivalent (a) guest series D2D2+–D6D2+ and D5+ or (b) guest series 1D12+–5D52+ and ADA2+. | |
In contrast, TA4 shows similar binding behaviors to guest series 1D12+–12D122+ (Fig. 3b and S22†). For 1D12+, all the peaks of TA4 appear to be broadened. A slow exchange is supported by the split of proton signals a + b of the NMR spectrum at room temperature and −20 °C (Fig. S33†). Aromatic protons appear as two peaks, again suggesting the existence of either conformer I or IV. With 2D22+, the TA4 appears as two sets of signals and one set is sharper than the other. For the guests with longer alkyl groups, the sharper signals become predominant. The peak patterns again suggest the existence of conformer I and/or IV. We compared the NMR spectra of these two guest series (Fig. S34†), and found that all the sharp peaks appear at almost the same chemical shifts and all the broadened peaks do too. These results strongly support conformers I and IV are mainly involved in the binding of these two guest series.
In order to distinguish between conformers I and IV, we resorted to 2D NMR spectroscopy. As shown in Fig. 4, the models of conformers I and IV require that: in conformer I, proton 2 is in close proximity to proton b but farther away from proton a; while in conformer IV, proton 2 is close to both protons a and b in space. Thus, ROESY NMR experiments were performed on D2D2+@TA4 and 1D12+@TA4 since both of them have separate signals for protons a and b. A Nuclear Overhauser Effect (NOE) was detected between protons 2 and b for D2D2+@TA4, but not between protons 2 and a, suggesting TA4 exists as conformer I. The ROESY NMR spectra of 6D62+@TA4 and ADA2+@TA4 (Fig. S41†) were similar to that of D2D2+@TA4. While for 1D12+@TA4, proton 2 had a NOE effect with both protons a and b, indicating the predominance of conformer IV. These assignments are in line with the ROESY NMR spectrum of D5+@TA4 in which both conformers I and IV are observed. It is also supported by the peak line-shapes in the NMR spectra: the NMR peaks of conformer IV are all broadened while the peaks of conformer I remain sharp, since conformer IV has a larger cavity with faster guest exchange kinetics. From guest D2D2+ to D5D2+, conformer I was gradually converted to conformer IV. With even longer linkers, this trend was reversed (Fig. S5 and S16†). From 1D12+ to 3D32+, conformer IV was gradually replaced by conformer I and another conformer. This is the conformational response of the host to the structural changes of guests.
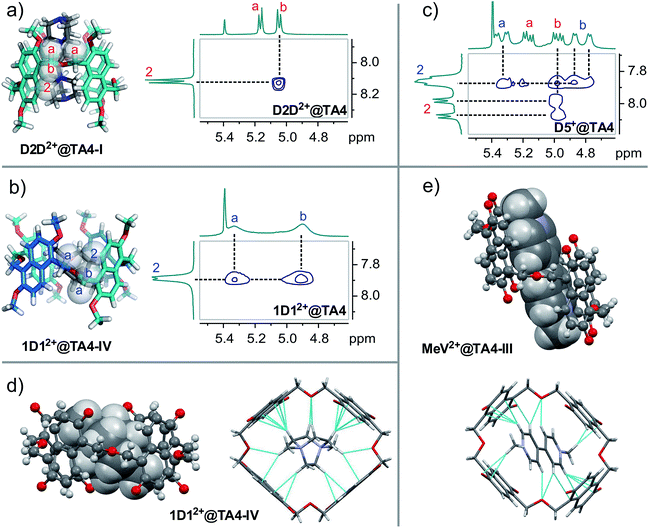 |
| Fig. 4 Energy-minimized structures and partial 1H, 1H-ROESY NMR spectra (500 MHz, CD2Cl2 : CD3CN = 1 : 1, 6.0 mM, 25 °C) of (a) D2D2+@TA4-I, (b) 1D12+@TA4-IV and (c) D5+@TA4. The protons a, b, a′, b′, 2′ and 2 in the models are rendered to show possible NOE contacts; single crystal structures of (d) MeV2+@TA4-III and (e) 1D12+@TA4-IV. Butyl groups are removed for viewing clarity. | |
For guests 4D42+–12D122+ (Fig. S22†), besides the major peaks assigned to conformer I, there are many small peaks which have similar intensity and should belong to a single conformer of TA4. This conformation can be assigned to conformer II, since conformer II has the lowest symmetry (C2) and should show eight doublets for its aromatic protons. This is more clearly seen in the case of ADA2+ (Fig. 3b and S35†). For all these guests, conformer II accounts for 45–55% of TA4 in the solutions.
Compared to the 1,4-diazabicyclo[2.2.2]octane (DABCO) guests, viologen MeV2+ is longer and thinner, and is reasonably expected to select a different host conformation. However, the guest is too thin and undergoes fast exchange at the NMR timescale. This results in broadened NMR signals (Fig. 5a) and thwarts assignment of the host conformation. Two measures were taken to circumvent this problem: (a) decreasing the temperature; (b) attaching sizable (but not too large) end groups. Both ways worked efficiently to slow down the exchange. At −20 °C, all the peaks become significantly sharpened (Fig. 5b). The peak pattern suggests conformer III (C2h symmetry) to be the selected conformation. For guest AVA2+ with bulky end groups (Fig. 5c), the conformer III is also the major structure but conformer II can be identified to account for 30% of TA4. Conformer III has a long and narrower cavity and fits perfectly to such thin guests. ROESY NMR of AVA2+@TA4 (Fig. S41†) supports this assignment and the assignments of I and IV as well: conformer III has both NOEs involved in conformers I and IV, and the NOEs are exactly the same as those observed in these two conformers.
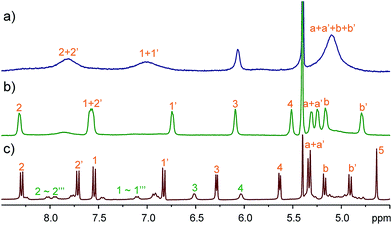 |
| Fig. 5 Partial 1H NMR spectra (CD2Cl2 : CD3CN = 1 : 1, 2.0 mM) of an equimolar mixture of TA4 and MeV2+ at (a) 25 °C (400 MHz) or (b) −20 °C (600 MHz) and (c) TA4 and AVA2+ (400 MHz, 25 °C). | |
Single-crystal structures
Single crystals of MeV2+@TA4 and 1D12+@TA4 were obtained by slow vapor diffusion of diethyl ether into the solutions in the 1
:
1 mixture of MeCN and CH2Cl2. The structures of TA4 (Fig. 4d and e and S54–S62†) were clearly shown to be in conformations III and IV for MeV2+@TA4 and 1D12+@TA4, respectively. This further consolidates the above assignments. For conformer IV, both enantiomers appear in pairs in the solid state (Fig. S61†). The binding mode is almost the same as those revealed by molecular modelling: 1D12+ is nested and tipped in the cavity; MeV2+ is threaded through and forms a [2]pseudorotaxane. In both structures, the oxygen atoms of CH2–O–CH2 are inwardly directed and multiple C–H⋯O hydrogen bonds27 are detected, supporting a non-innocent role for these linkers. In addition, C–H⋯π28 and cation⋯π interactions29 are also observed. Charge-transfer interactions were detected for MeV2+@TA4 by UV-vis spectroscopy (Fig. 6 and S63†).
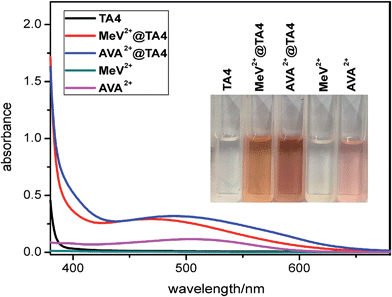 |
| Fig. 6 UV-vis absorption spectra (1.0 mM, 25 °C) of TA4, MeV2+-2PF6, AVA2+-2PF6, MeV2+-2PF6@TA4 and AVA2+-2PF6@TA4. Inset: photos of the above solutions. | |
Thermodynamic parameters
For all the guests shown above, one conformer or a combination of two or three conformers of TA4 is selected, and some examples are shown in Fig. 7. That is, different conformers have different binding affinities towards the same guest. The host also shows conformational responses to the structural change of guests. What are the thermodynamic consequences for such conformational responses? In order to answer this question, the association constants with selected guests were determined using isothermal titration calorimetry (ITC, see ESI†). A 1
:
1 binding stoichiometry for all these guests was obtained by ITC and ESI-MS. The data in Table 1 show that almost all the association constants are of the same order of magnitude, that is, 105 M−1, except for 1D12+ and D5D2+. From D2D2+ to D5D2+, the host conformation gradually changes from I to IV, and the association free energy only decreased by ca. 3 kJ mol−1. In view of the conformational equilibrium, the association free energy surely would undergo a larger decrease were the conformation fixed. From 1D12+ to 4D42+, the association free energy increased by 8 kJ mol−1, accompanied by a gradual conformational change from IV to a mixture of I and II. If the conformation is fixed, the increase should be smaller. In the case of MeV2+ and AVA2+, MeV2+ induces mainly conformer III; while AVA2+ selects 30% conformer II and 70% conformer III. However, their association free energies are not very different. Consequently, the conformational reservoir of the host shows conformational responses to different guests and thus maximizes the association free energy. Although the association free energies were changed only slightly, the binding entropies and enthalpies show much more drastic changes. In addition, the entropic contribution is large for all the guests, presumably due to the release of the solvent molecules in the host and the solvated guest during binding.
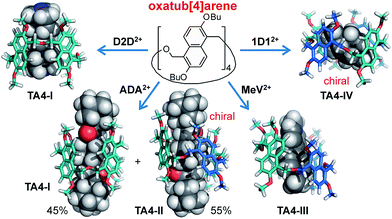 |
| Fig. 7 Summary of guest-selected predominance of one of the four conformers of TA4. Butyl groups in the models were abbreviated to methyl groups for viewing clarity. | |
Table 1 Association constants Ka to TA4 in 1,2-dichloroethane–MeCN (1
:
1) at 25 °C as determined by ITC
Guests |
K
a (105 M−1) |
ΔG (kJ mol−1) |
ΔH (kJ mol−1) |
−TΔS (kJ mol−1) |
1D12+
|
0.18 ± 0.03 |
−24.3 ± 0.7 |
3.10 |
−27.3 |
2D22+
|
1.44 ± 0.26 |
−29.4 ± 0.8 |
−7.30 |
−22.1 |
3D32+
|
4.57 ± 0.52 |
−32.3 ± 0.6 |
−13.0 |
−19.3 |
4D42+
|
4.30 ± 0.77 |
−32.2 ± 0.8 |
−11.9 |
−20.2 |
D2D2+
|
2.34 ± 0.63 |
−30.7 ± 0.9 |
−15.4 |
−15.2 |
D3D2+
|
1.56 ± 0.32 |
−29.6 ± 0.8 |
−8.7 |
−21.0 |
D4D2+
|
1.09 ± 0.19 |
−28.8 ± 0.8 |
−13.1 |
−15.6 |
D5D2+
|
0.75 ± 0.16 |
−27.8 ± 0.9 |
−8.43 |
−19.4 |
MeV2+
|
1.31 ± 0.19 |
−29.2 ± 0.7 |
−14.0 |
−15.2 |
AVA2+
|
1.82 ± 0.30 |
−30.0 ± 0.7 |
−15.3 |
−14.8 |
Conclusions
In summary, we report a new smart macrocyclic receptor, namely oxatub[4]arene. This macrocycle has four representative conformations, resulting from the flipping of naphthalene panels. Each conformer possesses a deep, well-defined cavity but their cavity sizes are different. Different guests select a single host conformer or a combination of conformers. All four conformers have been observed when different guests are applied, and characterized by 2D NMR and X-ray single crystallography. In the crystal structures, the oxygen atoms of CH2–O–CH2 are directed into the cavities and multiple C–H⋯O hydrogen bonds are detected, supporting a non-spectator role for the linkers. In addition, C–H⋯π, cation⋯π, and charge-transfer interactions are observed. Thermodynamically, the differences in association constants for different conformers to the same guest are greater than one order of magnitude. The host shows conformational responses to the structural changes of guests, which maximizes the association free energy compared to that expected for those macrocycles with a single cavity.
More generally, there are several consequences for the macrocycles with multiple interconvertible cavities: (a) the combination of several cavities into one macrocycle certainly expands its guest binding scope and improves the tolerance of structural change of guests. But low guest selectivity can also be expected; (b) at least three of the four conformations can be selected to predominate by appropriate guests for oxatub[4]arene. The alkyl groups on oxatub[4]arene are oriented in different patterns for different conformers. Thus, the guest-controlled transition among these conformations causes the allosteric change30 of the alkyl groups on the host. When replacing the alkyl groups with functional groups, this may be applied to the construction of molecular machines and devices and stimuli-responsive supramolecular materials; (c) the present macrocycle is not just another flexible macrocycle whose conformation can be tuned during binding through an induced-fit mechanism.31 Once the cavities are occupied by guests, these conformers cannot interconvert, since the flipping of naphthalene has to occur through the cavity which has little free space; (d) in the context of systems chemistry,32 the multiple conformations constitute a complex network, providing the possibility for complex functions. In summary, we believe this unique macrocyclic receptor further enriches the toolbox of supramolecular chemistry and may find wide applications in the near future.
Acknowledgements
This research is financially supported by the National Natural Science Foundation of China (No. 21302090, 21572097), Thousand Young Talents Program, South University of Science and Technology of China (FRG-SUSTC1501A-56) and the Shenzhen special funds for the development of biomedicine, internet, new energy, and new material industries (JCYJ20150331101823694).
Notes and references
-
(a)
Macrocyclic Chemistry: Current Trends and Future Perspectives, ed. K. Gloe, Springer, Dordrecht, 2005 Search PubMed;
(b)
F. Davis and S. Higson, Macrocycles: Construction, Chemistry and Nanotechnology Applications, Wiley, Chichester, 2011 Search PubMed.
-
(a)
J.-M. Lehn, Supramolecular Chemistry: Concepts and Perspectives, Wiley-VCH, Weinheim, 1995 Search PubMed;
(b)
J. W. Steed and J. L. Atwood, Supramolecular Chemistry, Wiley, Chichester, 2nd edn, 2009 Search PubMed.
-
(a) V. Balzani, A. Credi, F. M. Raymo and J. F. Stoddart, Angew. Chem., Int. Ed., 2000, 39, 3348–3391 CrossRef CAS;
(b) E. R. Kay, D. A. Leigh and F. Zerbetto, Angew. Chem., Int. Ed., 2007, 46, 72–191 CrossRef CAS PubMed;
(c) E. R. Kay and D. A. Leigh, Angew. Chem., Int. Ed., 2015, 54, 10080–10088 CrossRef CAS PubMed.
-
(a) B. Zheng, F. Wang, S. Dong and F. Huang, Chem. Soc. Rev., 2012, 41, 1621–1636 RSC;
(b) D.-S. Guo and Y. Liu, Chem. Soc. Rev., 2012, 41, 5907–5921 RSC;
(c) X. Ma and H. Tian, Acc. Chem. Res., 2014, 47, 1971–1981 CrossRef CAS PubMed.
-
(a) Z. Qi and C. A. Schalley, Acc. Chem. Res., 2014, 47, 2222–2233 CrossRef CAS PubMed;
(b) S. Dong, B. Zheng, F. Wang and F. Huang, Acc. Chem. Res., 2014, 47, 1982–1994 CrossRef CAS PubMed.
- X. Ma and Y.-L. Zhao, Chem. Rev., 2015, 115, 7794–7839 CrossRef CAS PubMed.
-
(a) M.-X. Wang, Chem. Commun., 2008, 4541–4551 RSC;
(b) M.-X. Wang, Acc. Chem. Res., 2012, 45, 182–195 CrossRef CAS PubMed.
-
(a) T. Ogoshi, S. Kanai, S. Fujinami, T.-A. Yamagishi and Y. Nakamoto, J. Am. Chem. Soc., 2008, 130, 5022–5023 CrossRef CAS PubMed;
(b) For reviews: M. Xue, Y. Yang, X.-D. Chi, Z.-B. Zhang and F. Huang, Acc. Chem. Res., 2012, 45, 1294–1308 CrossRef CAS PubMed;
(c) D.-R. Cao and H. Meier, Asian J. Org. Chem., 2014, 3, 244–262 CrossRef CAS PubMed;
(d) P. J. Cragg and K. Sharma, Chem. Soc. Rev., 2012, 41, 597–607 RSC;
(e) T. Ogoshi and T. A. Yamagishi, Eur. J. Org. Chem., 2013, 2961–2975 CrossRef CAS PubMed;
(f) H. Zhang and Y.-L. Zhao, Chem.–Eur. J., 2013, 19, 16862–16879 CrossRef CAS PubMed;
(g) N. L. Strutt, H. Zhang, S. T. Schneebeli and J. F. Stoddart, Acc. Chem. Res., 2014, 47, 2631–2642 CrossRef CAS PubMed;
(h) C. Li, Chem. Commun., 2014, 50, 12420–12433 RSC;
(i) M.-C. Xia and Y.-W. Yang, Progr. Chem., 2015, 27, 655–665 Search PubMed;
(j) Z. Zhang, Y. Luo, J. Chen, S. Dong, Y. Yu, Z. Ma and F. Huang, Angew. Chem., Int. Ed., 2011, 50, 1397–1401 CrossRef CAS PubMed.
-
(a) J. Svec, M. Necas and V. Sindelar, Angew. Chem., Int. Ed., 2010, 49, 2378–2381 CrossRef CAS PubMed;
(b) M. A. Yawer, V. Havel and V. Sindelar, Angew. Chem., Int. Ed., 2015, 54, 276–279 CrossRef CAS PubMed.
-
(a) H.-Y. Gong, B. M. Rambo, E. Karnas, V. M. Lynch and J. L. Sessler, Nat. Chem., 2010, 2, 406–409 CrossRef CAS PubMed;
(b) B. M. Rambo, H.-Y. Gong, M. Oh and J. L. Sessler, Acc. Chem. Res., 2012, 45, 1390–1401 CrossRef CAS PubMed.
-
(a) S. Lee, C.-H. Chen and A. H. Flood, Nat. Chem., 2013, 5, 704–710 CrossRef CAS PubMed;
(b) A. Singharoy, B. Venkatakrishnan, Y. Liu, C. G. Mayne, S. Lee, C.-H. Chen, A. Zlotnick, K. Schulten and A. H. Flood, J. Am. Chem. Soc., 2015, 137, 8810–8818 CrossRef CAS PubMed.
-
(a) S. T. Schneebeli, M. Frasconi, Z. Liu, Y. Wu, D. M. Gardner, N. L. Strutt, C. Cheng, R. Carmieli, M. R. Wasielewski and J. F. Stoddart, Angew. Chem., Int. Ed., 2013, 52, 13100–13104 CrossRef CAS PubMed;
(b) Z. Liu, G. Liu, Y. Wu, D. Cao, J. Sun, S. T. Schneebeli, M. S. Nassar, C. A. Mirkin and J. F. Stoddart, J. Am. Chem. Soc., 2014, 136, 16651–16660 CrossRef CAS PubMed.
-
(a) J. C. Barnes, M. Juríček, N. L. Strutt, M. Frasconi, S. Sampath, M. A. Giesener, P. L. McGrier, C. J. Bruns, C. L. Stern, A. A. Sarjeant and J. F. Stoddart, J. Am. Chem. Soc., 2013, 135, 183–192 CrossRef CAS PubMed;
(b) M. Juríček, J. C. Barnes, E. J. Dale, W.-G. Liu, N. L. Strutt, C. J. Bruns, N. A. Vermeulen, K. Ghooray, A. A. Sarjeant, C. L. Stern, Y. Y. Botros, W. A. Goddard III and J. F. Stoddart, J. Am. Chem. Soc., 2013, 135, 12736–12746 CrossRef PubMed;
(c) E. J. Dale, N. A. Vermeulen, A. A. Thomas, J. C. Barnes, M. Juríček, A. K. Blackburn, N. L. Strutt, A. A. Sarjeant, C. L. Stern, S. E. Denmark and J. F. Stoddart, J. Am. Chem. Soc., 2014, 136, 10669–10682 CrossRef CAS PubMed.
- Y. Chun, N. J. Singh, I. C. Hwang, J. W. Lee, S. U. Yu and K. S. Kim, Nat. Commun., 2013, 4, 1797 CrossRef PubMed.
-
(a) H. Chen, J. Fan, X. Hu, J. Ma, S. Wang, J. Li, Y. Yu, X. Jia and C. Li, Chem. Sci., 2015, 6, 197–202 RSC;
(b) J. Zhou, G. Yu, L. Shao, B. Hua and F. Huang, Chem. Commun., 2015, 51, 4188–4191 RSC;
(c) J. Ma, H. Deng, S. Ma, J. Li, X. Jia and C. Li, Chem. Commun., 2015, 51, 6621–6624 RSC.
-
(a) Q.-H. Guo, Z.-D. Fu, L. Zhao and M.-X. Wang, Angew. Chem., Int. Ed., 2014, 53, 13548–13552 CrossRef CAS PubMed;
(b) Q.-H. Guo, L. Zhao and M.-X. Wang, Angew. Chem., Int. Ed., 2015, 54, 8386–8389 CrossRef CAS PubMed.
-
(a) C.-F. Chen, Chem. Commun., 2011, 47, 1674–1688 RSC;
(b) P. Xin, L. Zhang, P. Su, J.-L. Hou and Z.-T. Li, Chem. Commun., 2015, 51, 4819–4822 RSC;
(c) J.-H. Wang, H.-T. Feng and Y.-S. Zheng, Chem. Commun., 2014, 50, 11407–11410 RSC;
(d) H.-J. Zhou, Y.-S. Zhao, G. Gao, S.-Q. Li, J.-B. Lan and J.-S. You, J. Am. Chem. Soc., 2013, 135, 14908–14911 CrossRef CAS PubMed.
-
(a) J.-M. Lehn, Chem.–Eur. J., 1999, 5, 2455–2463 CrossRef CAS;
(b) P. T. Corbett, J. Leclaire, L. Vial, K. R. West, J.-L. Wietor, J. K. M. Sanders and S. Otto, Chem. Rev., 2006, 106, 3652–3711 CrossRef CAS PubMed;
(c) J.-M. Lehn, Chem. Soc. Rev., 2007, 36, 151–160 RSC;
(d)
J. N. H. Reek and S. Otto, Dynamic Combinatorial Chemistry, Wiley-VCH, Weinheim, 2010 Search PubMed;
(e) F. B. L. Cougnon and J. K. M. Sanders, Acc. Chem. Res., 2012, 45, 2211–2221 CrossRef CAS PubMed;
(f) J. Li, P. Nowak and S. Otto, J. Am. Chem. Soc., 2013, 135, 9222–9239 CrossRef CAS PubMed.
-
(a) Z. He, G. Ye and W. Jiang, Chem.–Eur. J., 2015, 21, 3005–3012 CrossRef CAS PubMed;
(b) G. Huang and W. Jiang, Progr. Chem, 2015, 27, 744–754 Search PubMed.
- G. Huang, Z. He, C.-X. Cai, F. Pan, D. Yang, K. Rissanen and W. Jiang, Chem. Commun., 2015 10.1039/c5cc06768e.
- For reviews:
(a) V. Böhmer, Angew. Chem., Int. Ed. Engl., 1995, 34, 713–745 CrossRef PubMed;
(b) A. Ikeda and S. Shinkai, Chem. Rev., 1997, 97, 1713–1734 CrossRef CAS PubMed;
(c) For recent examples: C. Talotta, C. Gaeta, Z. Qi, C. A. Schalley and P. Neri, Angew. Chem., Int. Ed., 2013, 52, 7437–7441 CrossRef CAS PubMed;
(d) C. Talotta, N. A. de Simone, C. Gaeta and P. Neri, Org. Lett., 2015, 17, 1006–1009 CrossRef CAS PubMed.
- B.-L. Poh, C. S. Lim and K. S. Khoo, Tetrahedron Lett., 1989, 30, 1005–1008 CrossRef CAS.
- G. D. Andreetti, V. Boehmer, J. G. Jordon, M. Tabatabai, F. Ugozzoli, W. Vogt and A. Wolff, J. Org. Chem., 1993, 58, 4023–4032 CrossRef CAS.
-
(a) P. E. Georghiou and Z. Li, Tetrahedron Lett., 1993, 34, 2887–2890 CrossRef CAS;
(b) P. E. Georghiou, Z. Li, M. Ashram, S. Chowdhury, S. Mizyed, A. H. Tran, H. Al-Saraierh and D. O. Millera, Synlett, 2005, 879–891 CrossRef CAS;
(c) A. H. Tran, D. O. Miller and P. E. Georghiou, J. Org. Chem., 2005, 70, 1115–1121 CrossRef CAS PubMed;
(d) T. A. AlHujran, L. N. Dawe and P. E. Georghiou, Org. Lett., 2012, 14, 3530–3533 CrossRef CAS PubMed;
(e) H.-A. Tran, J. Collins and P. E. Georghiou, New J. Chem., 2008, 32, 1175–1182 RSC.
-
(a) B. J. Shorthill, C. T. Avetta and T. E. Glass, J. Am. Chem. Soc., 2004, 126, 12732–12733 CrossRef CAS PubMed;
(b) C. T. Avetta, B. J. Shorthill, C. Ren and T. E. Glass, J. Org. Chem., 2012, 77, 851–857 CrossRef CAS PubMed.
- K. Cottet, P. M. Marcos and P. J. Cragg, Beilstein J. Org. Chem., 2012, 8, 201–226 CrossRef CAS PubMed.
- R. K. Castellano, Curr. Org. Chem., 2004, 8, 845–865 CrossRef CAS.
-
M. Nishio, M. Hirota and Y. Umezawa, The CH/π Interaction: Evidence, Nature, and Consequences, Wiley, Chichester, 1998 Search PubMed.
- D. A. Dougherty, Acc. Chem. Res., 2013, 46, 885–893 CrossRef CAS PubMed.
-
(a) C. Kremer and A. Lützen, Chem.–Eur. J., 2013, 19, 6162–6196 CrossRef CAS PubMed;
(b) P. C. Knipe, S. Thompson and A. D. Hamilton, Chem. Sci., 2015, 6, 1630–1639 RSC.
- D. E. Koshland Jr, Proc. Natl. Acad. Sci. U. S. A., 1958, 44, 98–104 CrossRef.
-
(a) R. F. Ludlow and S. Otto, Chem. Soc. Rev., 2008, 37, 101–108 RSC;
(b) J. R. Nitschke, Nature, 2009, 462, 736–738 CrossRef CAS PubMed;
(c) E. Mattia and S. Otto, Nat. Nanotechnol., 2015, 10, 111–119 CrossRef CAS PubMed.
Footnote |
† Electronic supplementary information (ESI) available: Experimental conditions and procedures, syntheses and compound characterizations, 1H, 13C and 2D NMR spectroscopic analyses, mass spectra, ITC titration data and analyses, and X-ray single-crystal structure data and analyses. CCDC 1D12+@TA4-IV, 1043635; MeV2+@TA4-III, 1043636. For ESI and crystallographic data in CIF or other electronic format see DOI: 10.1039/c5sc03251b |
|
This journal is © The Royal Society of Chemistry 2015 |