Sediment microbial fuel cells for wastewater treatment: challenges and opportunities
Received
25th January 2015
, Accepted 26th March 2015
First published on 27th March 2015
Abstract
Sediment microbial fuel cells (SMFCs) have been intensively investigated for the harvest of energy from natural sediment, but studies of their application for wastewater treatment mainly occurred in the past 2–3 years. SMFCs with simple structures can generate electrical energy while decontaminating wastewater. Most SMFCs used for wastewater treatment contain plants to mimic constructed wetlands. Both synthetic and real wastewaters have been used as substrates in SMFCs that achieved satisfactory performance in organic removal. SMFCs have also been scaled up from several litres to more than 150 L. To further develop this technology, identification of a suitable application niche is needed. Several challenges must be addressed, including more detailed analysis in energy production, consumption, and application, understanding the relationship between electricity generation and contaminant removal, selecting plants that will benefit electrode reactions, improving nutrients removal, and optimizing system configuration and operation. The potential applications of SMFCs for wastewater treatment include powering sensors to monitor treatment processes and enhancing the removal of specific contaminants by electricity generation.
Water impact
Sediment microbial fuel cells (SMFCs) can extract electrical energy from organic compounds in natural sediment. Wastewater treatment using SMFCs appears to be a relatively new application. It has been demonstrated that SMFCs can effectively treat wastewater while generating electricity. The gap in knowledge lies in insufficient understanding of the limitations of SMFCs. Among those limitations, low energy recovery due to SMFCs' large internal resistance suggests that SMFCs may not be able to produce massive energy from wastewater. However, a clear understanding of the limitation in energy production also opens the door for several potential applications, such as powering sensors to monitor treatment processes or enhancing the removal of specific contaminants with the aid of electricity generation.
|
Introduction
Suitable treatment of wastewater is important to human health and societal development, and the commonly applied wastewater treatment technologies based on aerobic treatment have a significant demand for energy. Thus, new treatment technologies with low energy consumption and possible recovery of valuable resources (e.g., energy and water) from wastewater are of strong interest. Among the newly developed concepts, microbial fuel cells (MFCs) appear to be very attractive because of direct electricity generation from organic compounds by taking advantage of microbial metabolism with solid electron acceptors or donors.1 In an MFC, microorganisms donate electrons generated from organic oxidation to an electrode (anode) and/or accept electrons from an electrode (cathode) to reduce the terminal electron acceptor.2 Electron flow, as a result of bioelectrochemical and electrochemical reactions, generates electrical current and power. In general, there are two types of MFCs: reactor MFCs and sediment MFCs.
Reactor MFCs are engineered systems (Fig. 1A) that are designed and operated for energy-efficient water/wastewater treatment or (after appropriate modification) production of specific compounds such as hydrogen gas (microbial electrolysis cells) and value-added compounds (microbial electrosynthesis cells). Wastewater treatment is a well-known function and objective for the development of reactor MFCs,1 and tremendous efforts have been made to understand and improve reactor MFCs from the aspects of microbiology, electrochemistry, materials, and configuration. The technology has been advanced with long-term operation of MFCs installed in wastewater treatment plants3 and scaled up to several hundred liters.4
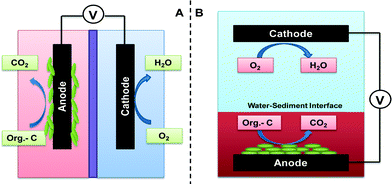 |
| Fig. 1 Schematics of reactor MFCs (A) and sediment MFCs (B). “Org.-C”: organic carbon. | |
Sediment MFCs (SMFCs), also referred to as benthic MFCs in some cases,5 are deployed in a natural system or where there is less engineering management (e.g., a constructed wetland). Unlike reactor MFCs that have a clear boundary between the anode and the cathode by using membranes or separators, SMFCs rely on a naturally occurring oxygen gradient to separate the anode and the cathode.6 To achieve that, the anode electrode is embedded in sediment where dissolved oxygen (DO) is depleted, while the cathode electrode is installed in the water phase with relatively higher DO (Fig. 1B). In the presence of plants, the anode electrode is placed near the rhizosphere to use organic compounds excreted from the roots;7 in some special cases, the cathode electrode can also be set up in the rhizosphere area to take advantage of the oxygen released from the plant roots.8
A major interest in SMFCs lies in the power supply for sensors in remote areas or deep waters. Because of the large internal resistance of those systems (e.g., several hundred ohms), insufficient supply of electron donors/acceptors, and unfavourable conditions (e.g., pH and temperature), the power output from SMFCs is generally very low (e.g., 10–50 mW cm−2), but those simple-structured bioelectrochemical systems can continuously generate electricity without a significant demand for maintenance, which is an obvious advantage in remote areas or deep water where replacement or maintenance of power sources is challenging and consumes a large amount of time and human power with associated cost. As a result, the development of SMFCs is rapid and there have been more reports of field/pilot tests of SMFCs than that of reactor MFCs. Examples of SMFC advancement include a demonstration of a SMFC deployed in the ocean to power a meteorological buoy9 and a SMFC installed in the deep ocean using cold seep as a fuel for power production.10 The majority of the efforts in SMFC development are focused on extracting electrons from organic compounds that are present in sediment, which have limited availability that restricts power production. Except for a few early studies that had a proactive supply of substrates to SMFCs,11–13 the major efforts to develop SMFCs for wastewater treatment occurred in the past 2–3 years. Given much understanding of both SMFCs and wastewater treatment, a combination of those two may provide an exciting solution if suitable application niches are identified; meanwhile, problems also occurred with the existing studies. In the following sections, we will review the existing studies that are specifically focused on SMFCs used for wastewater treatment (either real or synthetic wastewater) and provide our perspectives on both opportunities and challenges to further develop this technology.
SMFCs for wastewater treatment
SMFCs for wastewater treatment are applied through a combination of SMFCs and natural wastewater treatment systems. Biological wastewater treatment mimics microbial degradation of organic compounds occurring in nature. Thus, natural systems such as water ponds and wetlands have been used to treat wastewater, sometimes with modification (e.g., aeration). The combination creates a treatment approach that inherits the advantages of both technologies, such as energy production in SMFCs and less engineering management with a natural treatment system. There are also additional benefits generated from this combination. First, wastewater provides more organic compounds (electron source) for anode oxidation of a SMFC and helps improve electricity generation; second, the electricity-generating process could stimulate removal of some recalcitrant compounds contained in wastewater or sediment;5 and third, the generated electricity may be applied to offset energy consumption by the treatment system or to power sensors for continuous and automatic monitoring of the treatment system. Table 1 summarizes the major characteristics and findings of the studies of the SMFCs used for wastewater treatment. The data of energy recovery (kWh m−3)14 were estimated from the power output, the volume of wastewater treated, and the hydraulic retention time (HRT) obtained from the literature (when available). One can see that SMFCs could achieve >90% removal of COD at a low organic loading rate less than 0.2 kg m−3 d−1. Those SMFCs are roughly grouped in two categories, depending on the use of plants.
Table 1 Major characteristics and findings in the studies of SMFCs used for wastewater treatment. Some data represent high-end values when multiple values are reported
Wastewater |
HRTa (day) |
V
(L) |
Flow pattern |
Plant |
CODinc (kg m−3 d−1) |
R
COD
(%) |
R
N
(%) |
CEg (%) |
P
max
(W m−3) |
Energyi (kWh m−3) |
Ref. |
Hydraulic retention time.
Liquid volume of the SMFC.
COD loading rate.
COD removal efficiency.
Total nitrogen removal.
Ammonia removal.
Coulombic efficiency.
The maximum power density.
Normalized energy recovery, estimated from the data in the literature.
|
Synthetic |
26.7 |
4.8 |
Horizontal |
None |
<0.001 |
— |
— |
— |
— |
— |
15
|
Aquaculture |
— |
9.5 |
Vertical |
None |
— |
84.4 |
95.3 |
— |
0.002 |
— |
16
|
Aquaculture |
— |
16.8 |
Vertical |
None |
— |
96 |
84 |
0.45 |
0.038 |
— |
17
|
Diary |
19 |
75.7 |
Batch |
None |
<0.01 |
93 |
— |
— |
0.019 |
0.0087 |
18
|
Synthetic |
3 |
1.4 |
Vertical |
Ipomoea aquatica
|
0.08 |
>95 |
— |
0.6–10.5 |
0.309 |
0.0222 |
19
|
Synthetic |
2 |
12.4 |
Vertical |
Ipomoea aquatica
|
0.10 |
94.8 |
90.8 |
0.4–1.3 |
0.071 |
0.0034 |
20
|
Synthetic |
2 |
2.3 |
Vertical |
Canna indica
|
2.24 |
65 |
|
<0.1 |
0.028 |
0.0014 |
21
|
Swine |
1.25 |
3.7 |
Vertical |
Phragmites australis
|
0.85 |
76.5 |
49 |
0.1–0.6 |
0.042 |
0.0014 |
22
|
Synthetic |
3.2 |
150 |
Horizontal |
Phragmites australis
|
0.18 |
95 |
|
0.3–0.5 |
0.094 |
0.0072 |
23
|
Swine |
1 |
8 |
Vertical |
Phragmites australis
|
0.58 |
80 |
75f |
— |
0.268 |
0.0064 |
24
|
Synthetic |
3 |
12.4 |
Vertical |
Ipomoea aquatica
|
0.06 |
85.7 |
— |
— |
0.302 |
0.0214 |
25
|
Municipal |
2.5 |
123 |
Horizontal |
Phragmites australis
|
0.03 |
81.6 |
85f |
— |
<0.001 |
<0.0001 |
26
|
Synthetic |
1–4 |
12.4 |
Vertical |
Ipomoea aquatica
|
0.10 |
85.7 |
— |
0.5 |
0.852 |
0.0613 |
27
|
The SMFCs without plants are just like those conventional SMFCs deployed in the lake or ocean, except the active supply of wastewater. The SMFCs were studied for aquaculture water treatment with observed effects of operating parameters on system performance.17 The wastewater supply in a surface flow mode will have input organic compounds existing in both sediment and liquid phases, and the presence of organics in the liquid phase creates two problems: (1) some electrons will not be able to be used for electricity generation, because the anode electrode is usually located in the sediment; and (2) those organics will stimulate the growth of heterotrophic bacteria on the cathode electrode, which will decrease the cathode performance by competing for DO and covering the electrode surface to preclude oxygen transfer. To address the above problems, a SMFC with multiphase floating electrodes was developed and investigated for electricity generation and organic removal.15 This SMFC contained a middle (anode) electrode that could use organic compounds in the liquid phase, and improved electricity generation was observed compared with conventional SMFCs or a floating-type MFC without middle electrodes. Another challenge for SMFC operation is the supply of electron acceptors, which is oxygen in most cases. Active aeration can provide sufficient DO,16 but it also requires significant energy consumption. Considering that SMFCs generate electrical energy, aeration may be provided by using self-generated energy, and this can be realized by incorporating an appropriately designed power management system. It was demonstrated that an aeration pump could be activated for a short period of time after about 20 min of operation of the SMFC, and this aeration improved organic removal by 21% for artificial wastewater or 54% for dairy wastewater.18 The improved treatment may benefit from increased DO by intermittent aeration and the consequent agitation of water that better distributes organic substrates for biodegradation and increases reaeration with atmospheric oxygen.
Incorporating plants into SMFCs create systems similar to constructed wetlands, which have been used for wastewater treatment for a long time.28 Those systems are also called “CW–MFC” (constructed wetland–microbial fuel cells) in some studies. Plants have a number of functions in those systems, such as providing substrates for bacterial attachment and biofilm formation, supplying carbon to microorganisms, uptake of some contaminants (e.g., nutrients), and regulating water flow in the wetland. There are two major plants used in SMFCs, Ipomoea aquatica and Phragmites australis, both of which have been applied and/or investigated for phytoremediation of wastewater in constructed wetlands. It has been demonstrated that the use of plants could significantly improve electricity generation, and the improvement was likely related to the increased DO excreted by the plant roots.20,25 The presence of plants also affects microbial communities in the anode and the cathode: microbial density in the cathode zone generally increased with the population of plants, possibly benefiting from plant roots that support microbial growth and biofilm formation; however, in the anode, the (same) researchers observed increased microbial population of several major bacteria (including Geobacter sulfurreducens that is a proven electricigenic bacterium) in one study20 while no significant change in another work.25 Therefore, it is not conclusive how plants could affect the anode community; one hypothesis is that the improved cathode reaction with plants may stimulate the population of anode electricigenic bacteria via electron demand. On the other hand, the influence on the cathode community is obvious, and further investigation can explore whether bacteria that can catalyze oxygen reduction reaction are also promoted by plants.
Wastewater is usually fed into constructed wetlands via two hydrological patterns, surface flow and subsurface flow.28 All the SMFC systems containing plants adopted subsurface flow, and most of them had vertical flow (upflow) of the influent (Table 1). The surface flow pattern is not favourable for SMFC application, likely because organic substrates cannot easily reach an anode electrode that is embedded in sediment. Subsurface flow introduces organics to the anode electrode first, and then the treated effluent flows to the cathode electrode, thereby creating a relatively effective separation between the two electrodes (or two environments, anaerobic and aerobic). To increase DO in the cathode region, the researchers examined the combined upflow (anode) and downflow (cathode) in a SMFC and observed the increased power output and nitrogen removal.24 However, such an arrangement decreased organic removal, compared with other SMFCs with an upflow pattern, and might promote the growth of heterotrophic bacteria with organic flux on the cathode that will compete for oxygen with the cathode reaction and restrict autotrophic bacteria that may help catalyze oxygen reduction reaction. Vertical upflow can be easily applied in small-scale SMFCs, which are usually made in a tubular column; however, vertical flow in a constructed wetland is usually in a downflow pattern, because of hydraulic flow based on gravity for less energy consumption by the pumps. For the large-scale SMFCs that were reported, a horizontal flow pattern was used for the wastewater supply.23,26 In a 150 L scale SMFC (Fig. 2), it was found that the organic input significantly affected the system performance in several aspects: the DO in the cathode zone decreased from 2 to 0 mg L−1 when the COD concentration increased from 250 to 1100 mg L−1, and consequently electricity generation followed the same trend as that of DO.23 These results confirm our previous statement that the overload of organic compounds in the cathode zone can negatively affect the cathode reaction through stimulating heterotrophic bacteria to compete for DO (via aerobic degradation of organics) and block DO transfer (with biofilm covering the cathode electrode).
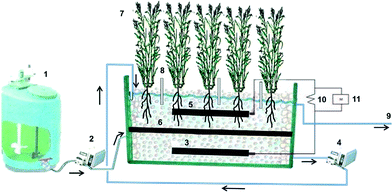 |
| Fig. 2 A horizontal subsurface constructed wetland–SMFC system. Reproduced with permission from ref. 23 | |
Challenges and opportunities
SMFCs have been studied intensively in the past decade, but their application for wastewater treatment is limited. The limited studies have demonstrated the feasibility of this application, with both challenges and opportunities to address. A key to success is to identify the suitable application niche for this technology, and to achieve that, several critical factors/challenges must be well addressed and understood.
Energy balance
An energy balance can reflect whether a SMFC is energy positive or negative:
where Enet is the net energy of a SMFC, determined by the difference between energy production (Ep) and energy consumption (Ec). Ep is the generation of electrical energy, affected by various factors such as substrates, operating conditions, and the presence of plants (whose role in electricity generation is yet to be determined); Ec may involve energy consumed by pumps and/or aeration. Unfortunately, most studies report only power density and the data of energy production are generally not available. A new energy parameter, normalized energy recovery (NER) that was previously proposed,14 was used here to express energy production (Table 1). If we use the highest energy recovery of 0.0613 kWh m−3 and treatment capacity of 250 m3 wastewater per day (for a small community of a few hundred people) as an example, the SMFC would generate about 15 kWh. Regarding energy consumption by SMFCs, wastewater flow by gravity will avoid energy consumption by the feeding pump, but recirculation or cathode aeration that was employed in some studies would create an energy demand. Therefore, evaluation of energy production in those systems should be appropriately conducted by considering energy consumption. If a SMFC can generate a positive Enet (energy production is more than energy consumption), then the next question will be how to use this energy. Powering intermittent aeration with this energy is a promising approach,18 and the long-term behaviour of aeration pumps in a continuously operated SMFC needs to be examined. It will also be practical to power wireless sensors to monitor the key parameters of a wastewater treatment process. Other applications include powering LED lights or other low-power electronics.
Relationship between electricity generation and substrate degradation
The Coulombic efficiency (CE) of the SMFCs used for wastewater treatment is generally very low (<1%, Table 1), suggesting that the electricity-generating process contributes very little to the degradation of bulk organic compounds. However, it is also reported that SMFCs may stimulate the removal of some specific compounds.5 For example, a plant-based SMFC achieved 15% more decolorization under close-circuit conditions (electricity generation) than that under open-circuit conditions (no electricity generation).25 Another example is to use SMFCs for nitrate removal from the treated effluent, in which electricity generation improved nitrate removal by 10%, mainly because the SMFC was able to extract electrons from organics in sediment for nitrate reduction in the liquid phase (unpublished data). Those findings encourage further investigation of using electricity generation as a driving force to improve degradation of specific compounds in wastewater. To achieve that goal, SMFCs should be operated for maximum current generation, e.g., under very small external resistance or short-circuit conditions, which will lead to little energy recovery. Although the trade-off between energy recovery and removal of recalcitrant compounds should be further analyzed, given generally low energy production in SMFCs and environmental benefits of removing recalcitrant compounds, it could be more beneficial to have SMFCs operated for high current generation for degradation purpose in those cases.
Plants
The selection of plants for SMFCs largely depends on the region where the study is conducted and the availability of the plants. It will be interesting to understand how the installation position and density of plants will maximize the electrode performance. Plants can physically affect water flow and increase oxygen concentration, thereby influencing the distribution of electron donors/acceptors. Rhizospheric microorganisms and the cathode community in the presence of plants should be further analyzed to understand their role in catalyzing oxygen reduction.
Nutrient removal
Several studies have reported effective nitrogen removal in SMFC when treating wastewater.16,17,20,22,24 The removal was almost independent from the electricity-generating process and mainly through nitrification that reduces ammonium but accumulates nitrate. Further investigation should link electricity generation to nitrogen removal, for example, accelerated ammonium transport from the anode to the cathode by electricity generation or bioelectrochemical nitrate reduction on the cathode.29 Phosphorus removal appears to have a weak link to electricity generation, which may elevate the catholyte pH to assist phosphate precipitation.
Optimized system design and operation
The absence of an effective separator and certain flow patterns in a SMFC often expose its cathode to electron donors (e.g., organics) and its anode to electron acceptors (e.g., oxygen), thereby decreasing efficiency of electrochemical reactions. Decreasing the gap between two electrodes, for the purpose of lowering internal resistance, may further increase the chance of crossover of electron donors/acceptors between two environments. System optimization may consider the use of an ion exchange membrane to improve the separation and thus electricity generation, though at a cost of higher capital investment. Low cost cathode catalysts such as nitrogen-doped activated carbon30 could be applied to improve the cathode reaction. Another factor for a better cathode reaction is to increase DO in the cathode zone. As previously discussed, intermittent aeration powered by SMFCs could be an option, and the aeration may also be powered by other renewable energy such as solar or wind energy if available. The DO may also be increased by using photosynthetic organisms such as algae, which will also help remove nutrient compounds;31 algae separation or harvesting from the final effluent will remain a challenge. The flow pattern is another key factor to be optimized. Vertical flow may achieve effective delivery of substrates to the anode without significant influence on the cathode, while horizontal flow will be more suitable for large-scale systems; if gravity allows, one may consider combined vertical and horizontal flow.
Conclusions
We must admit that SMFCs do not generate much electrical energy because of their inherent limitations (e.g., large internal resistance). Many data were obtained from synthetic wastewater and under laboratory conditions. Based on our experience, the real wastewater and field conditions will result in much lower energy production. Therefore, it may not be realistic to expect massive energy production from the SMFCs used for wastewater treatment. However, a clear understanding of the SMFCs' limitation in energy production does not preclude their application for wastewater treatment; instead, it can help us to identify appropriate application niches. Given the advantages and limitations of SMFCs, there could be two potential applications for wastewater treatment that can be realized in the near future: first, SMFCs may be used to generate energy to power sensors that monitor treatment systems, and this application does not require very large-scale SMFCs; and second, SMFCs may be applied to enhance the removal of some specific contaminants by electricity generation. Other applications can also be explored with further understanding and advancement of SMFC systems.
Acknowledgements
This work is supported by a grant from National Science Foundation (#1348424). We would like to thank Dr. Yaobin Lu (Virginia Tech) for his help with the graphs.
References
- W.-W. Li, H.-Q. Yu and Z. He, Energy Environ. Sci., 2014, 7, 911–924 CAS.
- B. E. Logan, B. Hamelers, R. A. Rozendal, U. Schroder, J. Keller, S. Freguia, P. Aelterman, W. Verstraete and K. Rabaey, Environ. Sci. Technol., 2006, 40, 5181–5192 CrossRef CAS.
- F. Zhang, Z. Ge, J. Grimaud, J. Hurst and Z. He, Environ. Sci. Technol., 2013, 47, 4941–4948 CrossRef CAS PubMed.
- Y. Feng, W. He, J. Liu, X. Wang, Y. Qu and N. Ren, Bioresour. Technol., 2014, 156, 132–138 CrossRef CAS PubMed.
- W.-W. Li and H.-Q. Yu, Biotechnol. Adv., 2015, 33, 1–12 CrossRef CAS PubMed.
- D. R. Lovley, Nat. Rev. Microbiol., 2006, 4, 497–508 CrossRef CAS PubMed.
- A. Kouzuma, N. Kaku and K. Watanabe, Appl. Microbiol. Biotechnol., 2014, 98, 9521–9526 CrossRef CAS PubMed.
- Z. Chen, Y.-C. Huang, J.-H. Liang, F. Zhao and Y.-G. Zhu, Bioresour. Technol., 2012, 108, 55–59 CrossRef CAS PubMed.
- L. M. Tender, S. A. Gray, E. Groveman, D. A. Lowy, P. Kauffman, J. Melhado, R. C. Tyce, D. Flynn, R. Petrecca and J. Dobarro, J. Power Sources, 2008, 179, 571–575 CrossRef CAS PubMed.
- M. E. Nielsen, C. E. Reimers, H. K. White, S. Sharma and P. R. Girguis, Energy Environ. Sci., 2008, 1, 584–593 CAS.
- Z. He, H. Shao and L. T. Angenent, Biosens. Bioelectron., 2007, 22, 3252–3255 CrossRef CAS PubMed.
- Z. He, J. J. Kan, Y. B. Wang, Y. L. Huang, F. Mansfeld and K. H. Nealson, Environ. Sci. Technol., 2009, 43, 3391–3397 CrossRef CAS.
- J. An, D. Kim, Y. Chun, S. J. Lee, H. Y. Ng and I. S. Chang, Environ. Sci. Technol., 2009, 43, 1642–1647 CrossRef CAS.
- Z. Ge, J. Li, L. Xiao, Y. Tong and Z. He, Environ. Sci. Technol. Lett., 2014, 1, 137–141 CrossRef CAS.
- J. An, H. Moon and I. S. Chang, Environ. Sci. Technol., 2010, 44, 7145–7150 CrossRef CAS PubMed.
- T. K. Sajana, M. M. Ghangrekar and A. Mitra, Aquacult. Eng., 2013, 57, 101–107 CrossRef PubMed.
- T. K. Sajana, M. M. Ghangrekar and A. Mitra, Aquacult. Eng., 2014, 61, 17–26 CrossRef PubMed.
- T. Ewing, J. T. Babauta, E. Atci, N. Tang, J. Orellana, D. Heo and H. Beyenal, J. Power Sources, 2014, 269, 284–292 CrossRef CAS PubMed.
- S. Liu, H. Song, S. Wei, F. Yang and X. Li, Bioresour. Technol., 2014, 166, 575–583 CrossRef CAS PubMed.
- S. Liu, H. Song, X. Li and F. Yang, Int. J. Photoenergy, 2013, 2013, 172010 Search PubMed.
- A. K. Yadav, P. Dash, A. Mohanty, R. Abbassi and B. K. Mishra, Ecol. Eng., 2012, 47, 126–131 CrossRef PubMed.
- Y. Zhao, S. Collum, M. Phelan, T. Goodbody, L. Doherty and Y. Hu, Chem. Eng. J., 2013, 229, 364–370 CrossRef CAS PubMed.
- J. Villasenor, P. Capilla, M. A. Rodrigo, P. Canizares and F. J. Fernandez, Water Res., 2013, 47, 6731–6738 CrossRef CAS PubMed.
- L. Doherty, Y. Zhao, X. Zhao and W. Wang, Chem. Eng. J., 2014, 266, 74–81 CrossRef PubMed.
- Z. Fang, H.-L. Song, N. Cang and X.-N. Li, Bioresour. Technol., 2013, 144, 165–171 CrossRef CAS PubMed.
- C. Corbella, M. Garfí and J. Puigagut, Sci. Total Environ., 2014, 470–471, 754–758 CrossRef CAS PubMed.
- Z. Fang, H.-L. Song, N. Cang and X.-N. Li, Biosens. Bioelectron., 2015, 68, 135–141 CrossRef CAS PubMed.
- H. Wu, J. Zhang, H. H. Ngo, W. Guo, Z. Hu, S. Liang, J. Fan and H. Liu, Bioresour. Technol., 2015, 175, 594–601 CrossRef CAS PubMed.
- P. Kelly and Z. He, Bioresour. Technol., 2014, 153, 351–360 CrossRef CAS PubMed.
- B. Zhang, Z. Wen, S. Ci, S. Mao, J. Chen and Z. He, ACS Appl. Mater. Interfaces, 2014, 6, 7464–7470 CAS.
- L. Xiao and Z. He, Renewable Sustainable Energy Rev., 2014, 37, 550–559 CrossRef CAS PubMed.
|
This journal is © The Royal Society of Chemistry 2015 |