DOI:
10.1039/C4RA12054J
(Review Article)
RSC Adv., 2014,
4, 60581-60596
Recent advances in the synthesis, characterization, and biomedical applications of ultrasmall thiolated silver nanoclusters
Received
9th October 2014
, Accepted 28th October 2014
First published on 29th October 2014
Abstract
With ultrasmall particle sizes of ∼1 nm, thiolate-protected silver nanoclusters (or thiolated Ag NCs) have recently emerged as an attractive frontier of nanoparticle research because of their unique molecular-like properties, such as well-defined molecular structures, HOMO–LUMO transitions, quantized charging, and strong luminescence. Such intriguing physicochemical properties have made thiolated Ag NCs a new class of promising theranostic agents for a wide spectrum of biomedical applications, such as bioimaging, antimicrobial agents, and disease diagnostics and therapy. In turn, the promising applications of thiolated Ag NCs have also fuelled the cluster community to develop more efficient strategies to synthesize high-quality Ag NCs with well-defined size, structure, and surface. In this review article, we first survey recent advances in developing efficient synthetic strategies for thiolated Ag NCs, highlighting the underlying chemistry that makes the delicate control of their sizes and surfaces possible. In the second section, we discuss recent advances in characterization techniques for ultrasmall thiolated Ag NCs, including their physical, chemical, and biological properties. The emerging characterization techniques are central to the development of cluster chemistry. In the last section, we highlight some examples demonstrating the vast possibilities of thiolated Ag NCs for biomedical applications. We conclude this review article by pointing out some challenging issues related to thiolated Ag NCs, and hopefully these can encourage more concerted efforts on their study from the research communities of cluster chemistry, noble metal chemistry, biology, biomedicine, etc.
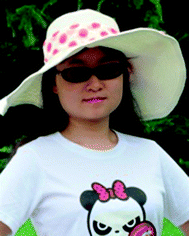 Kaiyuan Zheng | Kaiyuan Zheng received her BS degree from Peking University of China. She is currently a PhD student at the Department of Chemical and Biomolecular Engineering, National University of Singapore, under the supervision of Prof. Jianping Xie. Her current research interests are the synthesis and biomedical applications of metal nanoclusters. |
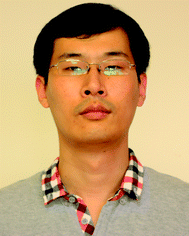 Xun Yuan | Xun Yuan obtained both his BE (2006) and ME (2009) degrees from Shandong University of Technology, China. He is currently a PhD student at the Department of Chemical and Biomolecular Engineering, National University of Singapore (NUS), under the supervision of Prof. Jianping Xie. He focuses his research on the synthesis and biological applications of luminescent metal nanoclusters. |
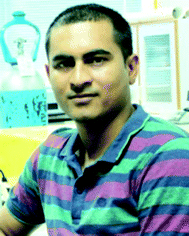 Nirmal Goswami | Dr Nirmal Goswami is currently a postdoctoral fellow in Prof. Jianping Xie’s group at the Department of Chemical and Biomolecular Engineering, National University of Singapore. He obtained his PhD (2014) in Chemistry from the S. N. Bose National Centre for Basic Sciences, India. He is interested in noble metal nanoclusters and their biomedical applications. |
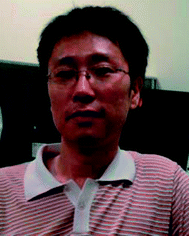 Qingbo Zhang | Dr Qingbo Zhang received his BS and MS from Tianjin University of China, and PhD in Chemical Engineering from the National University of Singapore. He is currently a postdoctoral research associate fellow in the Department of Chemistry, Rice University. His research interests include synthesis and surface modification of nanoparticles, exploration of their applications in the fields of healthcare, energy and environmental engineering, and evaluation of their environmental impact. |
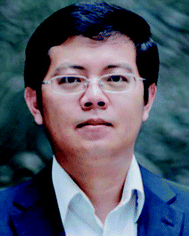 Jianping Xie | Dr Jianping Xie received his BS and MS from the Department of Chemical Engineering from Tsinghua University. He graduated with a PhD from the Singapore-MIT Alliance program. He joined National University of Singapore (NUS) as an Assistant Professor in 2010 and established the “Noble Metal Nanoclusters” research group. His major research interest is engineering subnanometer metal nanoclusters for biomedical and catalytic applications. |
1. Introduction
Metallic materials with particle sizes below 100 nm (or nanoscale metallic materials) exhibit distinctively different physical, chemical, and biological properties from their bulk materials.1–4 Further decreasing the particle size to a value comparable to the Fermi wavelength of electrons (∼1 nm for noble metals, such as gold (Au) and silver (Ag)), new physical and chemical properties emerge because the strong quantum confinement of free electrons starts to show effects in ultrasmall metal particles. One good example is noble metal nanoclusters (NCs).5–7 Metal NCs are ultrasmall particles with core sizes below 2 nm. Particles in this sub-2 nm size region possess discrete electronic states and unique geometric structures, exhibiting distinctively different physical and chemical properties from their larger counterparts – nanoparticles (NPs) with core sizes above 2 nm. As a result, ultrasmall metal NCs exhibit unique molecular-like properties, such as well-defined molecular structures,8–22 quantized charging,23–28 HOMO–LUMO transitions,8,23,29,30 molecular magnetism,31–33 molecular chirality,9,12,34–36 and strong luminescence.22,30,37–58 Such intriguing physicochemical properties of metal NCs, not having been seen in metal NPs (>2 nm) before, have attracted increasing interest from the research community for both basic and applied studies; and research on ultrasmall metal NCs has recently emerged as a frontier direction in the metal NP community.
Among the newly developed ultrasmall metal NCs, the NC family of silver is of particular interest, because Ag NCs not only possess the intriguing physicochemical properties of metal NCs, but also feature some unique properties pertaining to silver.38,59 For example, since ancient times, metallic silver has been known for its capability to combat infection. Recent studies showed that ultrasmall Ag NCs with core sizes of ∼1 nm exhibit superior antibacterial properties towards a wide spectrum of bacteria.60,61 The high antibacterial efficacy of ultrasmall Ag NCs, together with their unique chemical properties, could make them useful for disinfection applications in a variety of consumer products and medical devices. Furthermore, the unique physical properties of Ag NCs, such as their strong luminescence and ultrasmall size, provide good platforms to construct luminescent probes for bioimaging and sensing applications.38,59,62–67 Such applications have already surfaced in the community, showing the good potential of Ag NCs for biomedical applications.
The accessibility of high-quality Ag NCs with well-defined size, structure, and surface, is crucial to both basic and applied research in NC chemistry. Recent efforts from the cluster community have largely addressed this issue.68,69 For example, a number of efficient strategies have been recently developed for the synthesis of Ag NCs with tailorable physicochemical properties, and also of quantities large enough for practical applications.70–74 With delicate design of the synthetic chemistry, both bottom-up and top-down processes can be used to prepare high-quality Ag NCs. Besides the distinctively different physicochemical properties from large Ag NPs (>2 nm), the characterization techniques for Ag NCs are also different. Some emerging techniques, such as mass spectrometry and single crystal X-ray diffraction, have been recently used to characterize the physical and chemical properties of metal NCs, forming a central research direction in the development of cluster chemistry.63,68,73 Some of these characterization techniques are not suitable for metal NPs of relatively larger sizes. It is therefore a focus of this review article to provide a detailed discussion on the synthesis (with a specific focus on the underlying synthetic chemistry) and characterization (particularly focusing on some emerging characterization techniques pertaining to metal NCs) of functional Ag NCs.
We limit our discussion to one type of metal NCs – Ag NCs protected by thiolate ligands, or thiolated Ag NCs for short. Readers who are interested in other types of metal NCs, such as Au NCs and Ag NCs protected by other ligands like polymers, proteins, and DNA, may refer to several recent reviews on these topics.5,7,37–39,45,59,62,75–77 In this review article, we first survey recent advances in the development of efficient synthetic strategies for high-quality thiolated Ag NCs with well-defined size, structure, and surface, highlighting the underlying chemistry that can achieve good control in the formation of Ag NCs. In the second section, we discuss some recent developments of characterization techniques for Ag NCs (most of them can also be used for other metal NCs), with a particular focus on the working principles and key challenges pertaining to those characterization techniques for functional Ag NCs. In the last section, we highlight some emerging biomedical applications of thiolated Ag NCs, demonstrating some good designs of theranostic agents using thiolated Ag NCs. We then conclude this review article by pointing out some remaining issues (mostly challenging) in thiolated Ag NC research, and we hope that the synthetic chemistries and characterization techniques demonstrated here can be used to stimulate further development of new and effective synthetic protocols for high-quality Ag NCs, and also to further advance thiolated Ag NCs for more fundamental studies and practical applications.
2. Synthesis of thiolated Ag NCs
Compared to the synthesis of thiolated Au NCs, the synthesis of thiolated Ag NCs is more challenging because of their relatively poorer stability in solution under atmospheric conditions, especially in the presence of thiolate ligands (good etchants for atomic Ag). Therefore, more delicate control is required to synthesize Ag NCs with well-defined size and surface. There are some successful attempts; most of the reported protocols involve wet chemistry or solution chemistry, and only a few examples use solid state synthesis. The synthesis of thiolated Ag NCs can be roughly classified into two categories: bottom-up and top-down approaches, some of which have already been well-demonstrated in the synthesis of Ag NPs. In a typical bottom-up process, Ag ions (in the form of silver nitrate) are reduced by reducing agents, such as sodium borohydride (NaBH4), to form Ag atoms, which then nucleate and grow to form Ag NCs of desired sizes. In comparison, in a typical top-down process, relatively large Ag NPs (>2 nm) are used as Ag precursors, and they are carved into smaller sized Ag NCs in the presence of etching agents (or etchants), such as thiolate ligands. Both bottom-up and top-down approaches can be used to produce thiolated Ag NCs with well-defined and tailorable size, if the synthesis process can be delicately controlled.
The chemistries for the synthesis of thiolated Ag NCs via the bottom-up and top-down approaches are distinctively different. In particular, the key process in the bottom-up synthesis of Ag NCs is the reduction of Ag ions, where good control of the reduction kinetics is crucial for the formation of high-quality Ag NCs. By comparison, the key process in the top-down synthesis of Ag NCs is the digestion or etching of atomic Ag, where a good NC synthesis protocol requires a well-defined reaction environment that can facilitate a controllable etching reaction. In this section, we discuss the underlying chemistry involved in both processes for the formation of thiolated Ag NCs, highlighting some representative examples, with a particular focus on the concept demonstration rather than a comprehensive survey of the literature.
2.1. Bottom-up process
To date, the bottom-up process is the most widely used method to produce thiolated Ag NCs. With a delicate design of the synthetic chemistry, a bottom-up process is attractive for its high yield, short synthesis time, and facile reaction process. In a typical bottom-up process, thiolated Ag NCs are formed by reducing the Ag ions to metallic Ag in the presence of thiolate ligands. The thiolate ligands provide good protection for the newly formed Ag NCs in the reaction solution owing to the strong thiolate–Ag bond. However, if the reduction kinetics are not controlled in an effective manner, such as the use of a strong reducing agent (e.g., NaBH4), fast and poorly-controlled reduction kinetics often lead to the formation of polydisperse Ag NCs. Therefore, in order to obtain Ag NCs of a desired size, an etching or size-focusing process is required. The etching process can be performed either in the same reaction solution as the reduction (typically referred to as one-step synthesis) or in a different reaction solution (typically referred to as two-step synthesis).
2.1.1. Two-step synthesis. In a typical two-step protocol, the reduction of Ag ions to form Ag NC intermediates (often polydisperse) and the subsequent etching or digestion of the polydisperse Ag NC intermediates to form monodisperse Ag NCs are two separate steps, which involve two different reaction solutions. In the first step, the Ag(I)–thiolate complexes (typically formed by mixing Ag ions and thiolate ligands) are reduced to form Ag NC intermediates by a reducing agent. In the second step, the as-prepared Ag NC intermediates are used as precursors for size-focusing to form monodisperse Ag NCs via an etching process. An efficient two-step synthetic strategy requires good control of each step. Several efficient approaches have been recently developed to control the formation of Ag NC intermediates and the subsequent etching process for the formation of thiolated Ag NCs with well-defined size. We highlight some of these examples in this section.
(1) Delicate control of the NC intermediates – the size and structure matters. Good control of the NC intermediates is crucial to synthesize high-quality Ag NCs. The Ag NC intermediates, generally formed by the reduction of Ag(I)–thiolate complexes with a strong reducing agent like NaBH4, are the starting materials for the second step, which is the size-focusing or etching step. Therefore, the size and structure of the starting materials (NC intermediates) can affect the subsequent size-focusing step, leading to the formation of final Ag NCs with different quality. Some methods can be applied to control the size and structure of Ag NC intermediates. Here we highlight two such examples. The first one is the manipulation of the reduction kinetics of Ag(I)–thiolate complexes, and the second is the modification of the size/structure of Ag(I)–thiolate complexes before the formation of Ag NC intermediates.The reduction kinetics of Ag(I)–thiolate complexes are crucial for control of the size and structure of Ag NC intermediates. NaBH4, a strong reducing agent, is often used to reduce Ag(I)–thiolate complexes. This reaction is efficient, but the process is too fast and cannot be controlled in an effective manner in order to produce Ag NCs with well-defined size and structure. As a result, the NaBH4-reduction process often produces Ag NC intermediates with a broad size distribution. One way to improve the uniformity of the as-prepared NC intermediates is to slow down the reduction kinetics. Several reaction conditions can be used to decrease the reducing capability of NaBH4. For example, the reaction can be carried out at low temperature, and the reaction can also be designed to involve more than one phase; both conditions can help achieve relatively slower but better-controlled reduction kinetics for Ag NC formation.78 For example, by using this concept, a monodisperse thiolated Ag140 NC species has been successfully synthesized. On the other hand, solvents can also be used to reduce the reduction capability of NaBH4. For example, tetrahydrofuran (THF) has been used as the solvent to host the NC formation at 0 °C. In this case, use of the organic solvent can largely reduce the hydrolysis rate of NaBH4 and slow down its reduction rate, which leads to the formation of monodisperse thiolated Ag∼280 NCs.79 In addition, the solution pH and the concentration of reducing agents are another two reaction conditions that could be varied in order to adjust the reducing capability of NaBH4.
The modification of the reduction kinetics and also the formation of Ag(I)–thiolate complexes can be integrated into one protocol. One good example is the “cyclic reduction–decomposition” method, where both the size/structure of Ag(I)–thiolate complexes and their reduction kinetics were carefully controlled to synthesize thiolated Ag NCs with desired sizes.49,60,61 As illustrated in Fig. 1, the first step of this protocol is to mix the thiolate ligands (e.g., glutathione or GSH) with AgNO3 in water, forming Ag(I)–thiolate complexes with a broad size distribution. After that, a certain amount of NaBH4 is dissolved in NaOH (used to reduce the reducing capability of NaBH4 via inhibiting its hydrolysis rate), is introduced to reduce the Ag(I)–thiolate complexes to form Ag NC intermediates. The as-obtained Ag NC intermediates were polydisperse. They were not stable in water, and could be fully decomposed to Ag(I)–thiolate complexes in the presence of oxygen and excess thiolate ligands. Interestingly, the newly formed Ag(I)–thiolate complexes had a narrow size distribution and they were more resistant against NaBH4 reduction. As a result, a new type of Ag NC intermediates with a narrow size distribution was formed, which led to the formation of monodisperse Ag NCs after a subsequent size-focusing process. More interestingly, the as-synthesized thiolated Ag NCs showed strong luminescence. Similar to oxygen, which can assist the decomposition of Ag NCs in water, other oxidants such as H2O2 can also be used to facilitate the decomposition of Ag NC intermediates in the aqueous phase.80
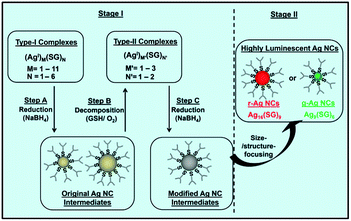 |
| Fig. 1 Schematic illustration of the cyclic reduction–decomposition process to produce luminescent GSH-protected Ag NCs in water. This method controls the formation of Ag NC intermediates. Reprinted with permission.60 Copyright 2013, Nature Publishing Group. | |
(2) Delicate control of the etching process – the etching environment matters. In a typical two-step protocol, the second step is the size-focusing of the as-prepared Ag NC intermediates to form monodisperse Ag NCs. Therefore, a good etching environment is needed to produce high-quality Ag NCs. Mild etching conditions are typically used to prepare good NC products. For example, a reversible phase transfer method was recently developed to create a mild etching environment to transform polydisperse and unstable Ag NC intermediates to monodisperse and stable Ag NCs.44,64 The process is illustrated in Fig. 2. This simple protocol can produce Ag NCs protected by different thiolate ligands. In this protocol, the polydisperse Ag NC intermediates are first prepared by reducing Ag(I)–thiolate complexes with a certain amount of NaBH4 in the aqueous phase. After that, the negatively-charged Ag NC intermediates are transferred to toluene by over-coating with a surfactant carrying a positively-charged head group (e.g., cetyltrimethylammonium bromide (CTAB)). In toluene, the Ag NC intermediates coated with CTA+ are slowly etched (or size-focused) to form monodisperse Ag NCs. Interestingly, after the synthesis, the monodisperse Ag NCs in toluene can be readily shuttled back to the aqueous phase.
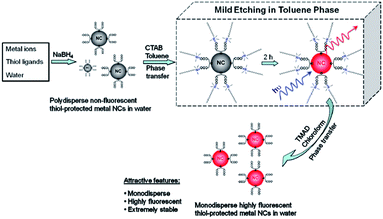 |
| Fig. 2 Schematic illustration of the use of a phase transfer cycle to produce luminescent thiolated metal NCs. This method creates a mild etching environment for Ag NC intermediates. Reprinted with permission.44 Copyright 2011, American Chemical Society. | |
2.1.2. One-step synthesis. Compared to the synthetic protocols involving two steps, synthetic protocols with only one step are generally more facile and can produce thiolated Ag NCs in a faster manner. In addition, the simplicity of such one-pot synthesis also translates to its good scalability. In a typical one-step process, the reduction of Ag(I)–thiolate complexes to form Ag NC intermediates and the size-focusing/etching of the as-formed NC intermediates occur simultaneously in the same reaction solution. Two reactions are involved in the formation of thiolated Ag NCs; the reduction of Ag(I)–thiolate complexes and the etching of metastable or newly-formed Ag NC intermediates. These two steps require careful control. Some efficient strategies aligned in this direction are highlighted in this section.
(1) Adjust the reaction conditions to create a mild reduction environment. NaBH4 is commonly used as the reducing agent to synthesize thiolated Ag NCs. However, the reduction kinetics with NaBH4 are generally fast, and such fast reaction, if without careful control, often leads to the formation of polydisperse Ag NCs. Meanwhile, another reaction also occurs during the reduction of Ag(I)–thiolate complexes, which is the etching of the newly formed Ag NCs back to Ag(I)–thiolate complexes by the excess thiolate ligands in the reaction solution. It is a reversible reaction, where a decrease in the reducing capability of NaBH4 and an increase in the etching capability of thiolate ligands may facilitate a rapid balancing of the reaction, leading to the formation of high-quality Ag NCs. Several methods can be used to slow down the reduction kinetics of NaBH4. The solution pH, the concentration of reducing agents, and the solvent used to host the reaction, are some reaction conditions that can be used to adjust the reducing capability of NaBH4.Among the above reaction conditions, adjusting the solution pH is one efficient way to control the reducing power of NaBH4 in the aqueous phase. For example, the introduction of a small amount of NaOH can slow down the reduction kinetics of NaBH4, resulting in the formation of thiolated Ag44 NCs (Fig. 3a).72 Similarly, a decrease in the concentration of NaBH4 can also slow down its reduction kinetics. For example, in a recent study, a very low concentration of NaBH4 was used to promote the formation of high-quality Ag NCs (Fig. 3b).81 In addition, adjusting the amount of reducing agents can also control the sizes of Ag NCs or NPs. For example, a low concentration of reducing agents helped synthesize a mixture of thiolated Ag4 and Ag5 NCs, which also showed intense red emission in solution.82 Solvents can also be used to control the reduction process. For example, using ethanol instead of water as the solvent (to host the reaction), a thiolated Ag7 NC was successfully prepared (Fig. 3c).70
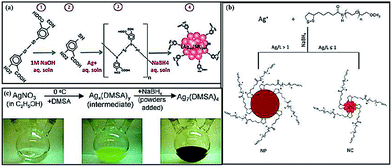 |
| Fig. 3 Schematic illustration of efficient protocols to synthesize thiolated Ag NCs by creating a mild reduction environment. (a) A synthesis protocol for thiolated Ag44 NCs by adjusting the solution pH. (b) A synthetic strategy for dihydrolipoic acid (DHLA)-protected Ag NCs by introducing a small amount of reducing agent. (c) Synthesis of Ag7(DMSA)4 (DMSA = dimercaptosuccinic acid) NCs in ethanol. Reprinted with permission.70,72,81 Copyright 2013, Royal Society of Chemistry. Copyright 2012, American Chemical Society. Copyright 2009, American Chemical Society. | |
Several of the above reaction conditions can also be combined to achieve a better control of the synthesis of thiolated Ag NCs. One good example is the synthesis of thiolated Ag44 NCs.68 In this reported protocol, the first step is the formation of Ag(I)–thiolate complexes by mixing an aqueous solution of AgNO3 with an excess ethanolic thiolate ligand. The reaction pH is then brought to ∼9.0 by adding a certain amount of CsOH, leading to the dissolution of the complexes. The solution pH is further changed to 12, at which a better protection of the newly formed Ag NCs by the thiolate ligands is made possible. In the final step, an aqueous solution of NaBH4 is added dropwise to reduce the Ag(I)–thiolate complexes to form atomically precise thiolated Ag44 NCs.
(2) Use mild reducing agents to create a mild reduction environment. Another efficient way to slow down the reduction kinetics for Ag NC formation is to replace NaBH4 with other mild reducing agents. Some mild reducing agents have been recently reported. Formic acid is one. It was used as a mild reducing agent (at an elevated temperature of 70 °C) to produce red-emitting thiolated Ag75 NCs (Fig. 4).83 In this protocol, the reaction temperature is adjusted to achieve optimized reduction kinetics for Ag NC formation. In addition, the increase in reaction temperature also increases the etching capability of thiolate ligands in solution, resulting in a better balance between the reduction and etching, which leads to the formation of thiolated Ag NCs with well-defined size.
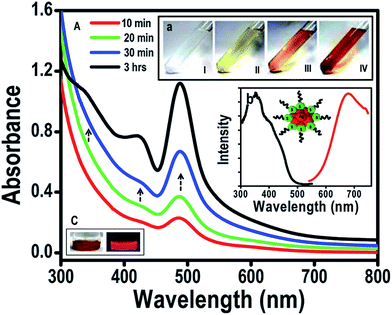 |
| Fig. 4 Synthesis of Ag75(SG)40 NCs by using formic acid as a reducing agent at high temperature. Reprinted with permission.83 Copyright 2012, Royal Society of Chemistry. | |
(3) Other methods to control the formation of Ag NCs. Some other techniques, such as light, ultra-sonication, and electricity, can also be used to create a mild reducing environment for the formation of Ag NCs. For example, sunlight has been used to reduce Ag ions to form Ag NCs in the organic phase.84 This protocol involves a photon-induced reduction, an autocatalytic nucleation, and a subsequent Ag NC growth process. More recently, ultrasound was also used to prepare thiolated Ag12 NCs.85 A possible mechanism is that the ultrasonic irradiation breaks the C–S bonds of the Ag(I)–thiolate complexes, generating free radicals that can be used to reduce Ag ions to Ag NCs. Another example is the formation of thiolated Ag5 and Ag6 NCs by using electricity.86Other methods, such as performing the reaction in the solid state or in a gel, can also be used to prepare thiolated Ag NCs. For example, a solid-state synthesis method was developed to produce red-emitting thiolated Ag9 NCs.71 This method can also synthesize thiolated Ag32 and Ag152 NCs.87,88 In addition, gels can also be used to host the synthesis of thiolated Ag NCs, and this can generate thiolated Ag25 NCs with strong red emission.89
2.2. Top-down process
Thiolated Ag NCs with well-defined size can also be produced via a top-down process, where a relatively larger Ag NP is etched to form small Ag NCs. Compared to the bottom-up process, there are fewer successful attempts reported involving the top-down process, since in general the latter is more time-consuming and often produces thiolated Ag NCs at lower yields. Such constraints can be partially addressed by optimizing the etching conditions, including etching time, reaction temperature, and the ratio of etchant to Ag precursors. In addition, an efficient top-down process could be a good complement to the bottom-up process.
In a top-down process, etching (typically with thiolate ligands) is a key reaction to transform large Ag NPs into monodisperse Ag NCs. Therefore, an efficient synthetic protocol requires a mild etching environment that makes possible a controlled formation of Ag NCs in the reaction solution. For example, an interfacial etching process (where the etching reaction involves two phases) was recently used to digest the as-prepared thiolated Ag NPs to form smaller Ag8 and Ag7 NCs.90 Similarly, two etchants, a nucleobase and thiolate ligands, were used in an interfacial etching protocol to produce a strong red-emitting Ag NC with a molecular weight of ∼7 kDa, as shown in Fig. 5.91 Another example is the synthesis of thiolated Ag38 NCs through the etching of large citrate-protected Ag NPs by excess thiolate ligands.92 The digestion of large Ag NPs to form small Ag NCs can also be achieved in harsh etching conditions, which often leads to the formation of thermodynamically stable Ag NC species. For example, at an elevated temperature, thiolated Ag NCs with different emissions were synthesized by a top-down process.93
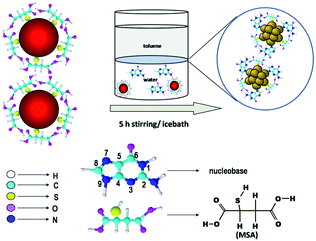 |
| Fig. 5 Schematic illustration of an interfacial etching protocol to produce Ag NCs. Reprinted with permission.91 Copyright 2009, Royal Society of Chemistry. | |
3. Characterization of thiolated Ag NCs
Thiolated Ag NCs are complicated supramolecules, typically consisting of several Ag atoms and a number of thiolate ligands. They can be denoted as Agn(SR)m where SR is the thiolate ligand, and n and m are specific integers. The physical, chemical, and biological properties of thiolated Ag NCs are influenced by their size, structure, and surface. Therefore, the characterization of such NC attributes is crucial to both basic and applied research of Ag NCs. Recently, a number of techniques have been applied to measure the physical, chemical, and biological properties of thiolated Ag NCs. Most of the techniques can also be used for other types of metal NCs. Some emerging characterization techniques are highlighted in this section.
3.1. Optical properties of thiolated Ag NCs
Optical spectroscopy is an important tool for the characterization of metal NCs because it provides useful information on the size and structure of metal NCs. Due to the ultrasmall size, the electrons in metal NCs are confined within a small volume, where the conduction and valence bands are separated, resulting in HOMO–LUMO transitions. Therefore, ultrasmall metal NCs show intriguing molecular-like properties and have discrete and size-dependent electronic transitions, including optical absorption, photoemission, and quantized charging. The optical properties of metal NCs are dictated by the cluster size, structure, and surface. In turn, the optical properties of metal NCs can also provide information about their size, structure, and surface.
(1) UV-vis absorption spectroscopy. In the last two decades, UV-vis absorption spectroscopy was extensively used to characterize Ag NPs due to their distinct optical absorption, caused by surface plasmon resonance (SPR).96–99 Similarly, ultrasmall Ag NCs also show distinct absorption peaks in the UV-vis region, and their optical absorption can be readily characterized by this instrument. It should be noted that the optical absorptions of Ag NPs and Ag NCs are distinctively different, with different origins and different peak locations.100 Based upon this, UV-vis absorption spectroscopy is often used as the first measure to differentiate Ag NCs from their larger counterparts, Ag NPs. In particular, the SPR peak of Ag NPs is typically located at ∼400 nm, whereas this peak is not seen in the absorption spectra of ultrasmall Ag NCs (<2 nm). In stark contrast, a thiolated Ag NC often shows several distinct absorption peaks in the UV-vis region. Such data can be used to confirm the successful synthesis of Ag NCs. For example, in a recent study, thiolated Ag NCs were successfully synthesized that showed multiple peaks in their absorption spectrum. However, upon heating the NC solution, the multiple absorption peaks merged into one single peak at ∼400 nm (a characteristic SPR peak of Ag NPs with particle sizes >2 nm), suggesting the formation of Ag NPs.101 In a separate study, UV-vis absorption spectra were used to confirm the transformation of small thiolated Ag75 NCs (∼0.9 nm in size) into large plasmonic Ag NPs of ∼2.9 nm.102UV-vis absorption spectroscopy also provides the first measure of size, structure, and surface for metal NCs because the optical absorption of metal NCs is dictated by these NC attributes. For example, Ag–SCH2CH2Ph NCs (here HSCH2CH2Ph refers to 2-phenylethanethiol) in toluene showed one absorption peak at ∼469 nm, while Ag–SPhF NCs (here HSPhF refers to 4-fluorothiophenol) in THF had two distinct peaks at 395 and 462 nm, and Ag-SG NCs (here SG refers to glutathione or GSH) in water had one peak at 478 nm.103 The data clearly suggest that the surface ligands on Ag NCs may affect their optical absorption. Similarly, the cluster size may also affect the optical absorption of Ag NCs. For example, thiolated Ag NCs with different numbers of Ag atoms (2 to 8) showed discrete absorption peaks in their UV-vis absorption spectra.104 More recently, the absorption spectra of Ag NCs with different sizes and thiolate ligands, such as Ag44(4-FTP)30, Ag55(PET)31, Ag75(PET)40, Ag114(PET)46, Ag152(PET)60, Ag202(BBS)70, Ag423(PET)105, and Ag530(PET)100, were studied (here 4-FTP, PET, and BBS refer to 4-flurothiophenol, 2-phenylethanethiol, and 4-(t-butyl)benzenethiol, respectively). As shown in Fig. 6a and b, thiolated Ag NCs with <114 Ag atoms displayed multiple absorption peaks, while those Ag NCs with >150 Ag atoms showed only one absorption peak at ∼460 nm, indicating that the emergence of metallicity occurred at about 150 Ag atoms.94
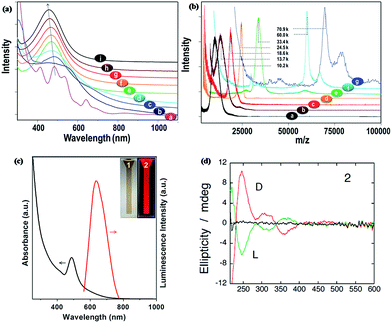 |
| Fig. 6 Optical properties of thiolated Ag NCs. (a) UV-vis absorption spectra of thiolated Ag44 [a], Ag55 [b], Ag∼75 [c], Ag∼114 [d], Ag152 [e], Ag∼202 [f], Ag∼423 [g], Ag∼530 [h] and Ag NPs [i]. (b) MALDI-TOF mass spectra of Ag55(PET)31 [a], ∼Ag75(PET)40 [b], ∼Ag114(PET)46 [c], Ag152(PET)60 [d], ∼Ag202(BBS)70 [e], ∼Ag423(PET)105 [f], and ∼Ag530(PET)100 [g]. (c) Optical absorption (black curve) and photoemission (red curve, λex = 489 nm) spectra of red-emitting thiolated Ag NCs in water. The inset shows photographs of the red-emitting Ag NCs in water under visible (item 1) and UV (item 2) light. (d) CD spectra of L-penicillamine-protected Ag NCs (green curve) and D-penicillamine-protected Ag NCs (red curve). Reprinted with permission.60,94,95 Copyright 2014, Royal Society of Chemistry. Copyright 2013, Nature Publishing Group. Copyright 2007, American Chemical Society. | |
Theoretical study is another good way to investigate the size-dependent optical absorption of thiolated Ag NCs. Time-dependent density functional theory (DFT) was used to simulate the optical absorption of thiolated Ag NCs. The data suggest that positions of the absorption peaks of Ag NCs are dictated by the cluster size and structure. In particular, the absorption spectra of tetrahedral Agn NCs (n = 10, 20, 35, 56, 84, and 120) were calculated, and these suggest that the distinct peaks of Agn NCs originate from their filled electronic shells.105 In addition, as the size of Ag NCs (or the value of n) increased, the absorption spectra shifted from molecular-like absorptions to those of plasmonic-like ones. Furthermore, the absorption peaks were red-shifted with the increase of cluster size.106 Interestingly, the optical absorption of the NCs was blue-shifted when their shape changed from octahedral to icosahedral, suggesting that the increase in the number of facets could lead to a blue shift of the optical absorption of metal NCs.
(2) Photoluminescence (PL) spectroscopy. Like organic molecules, thiolated Ag NCs are only stable at a particular ground state. If the Ag NCs are excited from the ground state, they will release extra energy before returning back to the ground state, which generates photoluminescence (PL). Recently, a number of Ag NCs have been shown to have strong PL (one example is shown in Fig. 6c).60 However, some fundamental issues related to the PL properties of Ag NCs are presently unclear. The PL of Ag NCs can be dictated by the cluster size, structure, and surface. For example, the PL spectrum of Ag152(SCH2CH2Ph)60 NCs (here HSCH2CH2Ph refers to 2-phenylethanethiol) shows two emission peaks,88 which arise from the core and the shell of the Ag NCs. In turn, the measurement of the PL properties of Ag NCs can also provide information about the cluster size and structure, because the PL properties are highly dependent on the size and structure of Ag NCs.
(3) Circular dichroism (CD). CD spectroscopy has been widely used to study the chiral properties and subtle conformational changes of organic molecules. It can also be used to characterize thiolated Ag NCs with some intriguing molecular-like properties, such as molecular chirality. Similar to organic molecules, the stereochemical structure of Ag NCs can also specifically absorb or deflect circularly polarized light. However, unlike organic molecules, most metal NCs do not show obvious chirality due to their relatively low symmetry. Some metal NCs can show chirality when they are coated with chiral molecules. For example, thiolated Ag NCs protected by D-captopril, D-glutathione, and D-cysteine show chiral properties.80 Similarly, the D/L-penicillamine-coated Ag NCs also showed chiral properties, where the enantiomers possessed an opposite CD spectrum (Fig. 6d).95 Further, non-chiral Ag NCs could become chiral when their surface ligand was replaced by the enantiopure D/L-penicillamine.107Since the chirality of Ag NCs is partially caused by the surface ligand, their chiral properties can be tuned by using different surface ligands. For example, the cluster structure and electronic state of Ag NCs protected by L-glutathione and N-acetyl-L-cysteine were found to be affected by the electronic field of the surface ligands.108 One can also increase the variability of chiral Ag NCs by using a mixture of two or more types of surface ligands. For example, mono- or mixed-chiral thiols have been used to synthesize Ag NCs with different chiralities.109
3.2. Size and molecular formula of thiolated Ag NCs
The particle size of metal NCs is their most important attribute, since their physical and chemical properties are highly dependent on the cluster size. Due to their ultrasmall size, the contribution of even one additional metal atom or thiolate ligand to the metal NCs is greatly amplified, resulting in extreme size sensitivity of the physical and chemical properties of metal NCs. Therefore, the determination of n and m in Agn(SR)m NCs (or the molecular formula of thiolated Ag NCs) is one of the central tasks in NC research. Several techniques can be used to estimate or precisely determine the size of thiolated Ag NCs.
(1) Transmission electron microscopy (TEM). TEM is one of the most powerful tools to characterize metal NPs, including their size, shape, and crystal structure.110 A TEM image is formed via the interaction of the electrons transmitted from the NPs. The size, shape, crystal structure, and defects of metal NPs can be readily revealed by TEM. Only the metal core, with a high electron density, can be seen under TEM. Compared to the characterization of metal NPs by TEM, the usability of TEM in metal NCs has been attempted less often, due to their ultrasmall size. Generally, TEM can only provide a size estimation for metal NCs (one example is shown in Fig. 7a), in particular for those NCs coated with a thick shell of protecting ligands (e.g., thiolated metal NCs).60 Precise determination of the size, cluster formula, and cluster structure cannot be achieved by conventional TEM, even with high-resolution TEM, if there is no delicate set-up and measurement design. There is one very recent successful attempt on thiolated Au NCs.111 In addition, metal NCs are very small, and the characterization of such tiny particles using TEM requires more careful sample preparation and measurement since the high-energy electron beam may damage the metal NCs and induce their fusion, which may provide false size information.
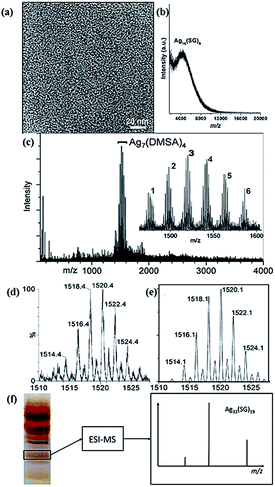 |
| Fig. 7 Size and molecular formula of thiolated Ag NCs. (a) A typical TEM image of thiolated Ag NCs. (b) MALDI-TOF mass spectrum of thiolated Ag16 NCs in water. (c–e) ESI mass spectra of thiolated Ag7 NCs. (f) A typical PAGE gel of mixed-sized thiolated Ag NCs. The Ag32(SG)19 compound is indicated (black box) and its structure was determined by ESI-MS. Reprinted with permission.60,70,73 Copyright 2013, Nature Publishing Group. Copyright 2009, American Chemical Society. Copyright 2012, American Chemical Society. | |
(2) Mass spectrometry. In the last two decades, matrix-assisted laser desorption ionization time-of-flight (MALDI-TOF) was used extensively to determine the size of metal NCs. MALDI-TOF can measure the molecular weight of thiolated metal NCs and also their molecular formula (one typical example is shown in Fig. 7b).60 The as-obtained molecular weight includes the metal core and the thiolate ligands. However, the strong laser radiation may break the Ag–S bond, or even some Ag atoms may dissociate from the metal core. Therefore, MALDI-TOF is usually combined with other, softer mass spectrometry techniques such as electrospray ionization (ESI) to determine the molecular formula of metal NCs.ESI mass spectrometry (ESI-MS) is the most powerful technique to determine the molecular weight and also the molecular formula of metal NCs. ESI-MS is a high-resolution analytical technique that can provide both qualitative (structure) and quantitative (molecular weight and formula) information for metal NCs. ESI can disperse Ag NC solutions by electrospray in a vacuum, and the charged NCs can be made to move under the electric field force. The introduction of the ionization source of the mass spectrometer can ionize the metal NCs to acquire positively- or negatively-charged clusters, which can then travel through the mass analyzer and arrive at different parts of the detector according to their mass/charge (m/z) ratio. Since molecules with different charges/weights travel at different speeds, through the time measurement, the molecular weight and the m/z peak can be obtained.
Compared to thiolated Au NCs, it is more challenging to determine the molecular formula of thiolated Ag NCs by using ESI-MS, because of their poorer stability. Fragments might be generated during the measurement, undermining the accuracy of the as-determined cluster formula. In addition, isotopes of Ag and S may also affect the accuracy of the measurement. There have been several successful attempts to determine the size of thiolated Ag NCs. Some examples are Ag44(MBA)30, Ag32(SG)19, Ag7(DMSA)4, and Ag16(SG)9 (here MBA and DMSA refer to mercaptobenzoic acid and dimercaptosuccinic acid, respectively) (Fig. 7c–e).60,68,70,73
(3) Polyacrylamide gel electrophoresis (PAGE). PAGE is a high resolution separation technique, which is extensively used in the biotechnology sector. More recently, PAGE has also been used to separate thiolated metal NCs with different sizes. It is a powerful technique, when combined with ESI-MS, to determine the cluster formula, since some synthetic protocols often produce metal NCs with mixed sizes. Such metal NCs can be efficiently separated by PAGE, and PAGE is therefore a powerful complementary technique to ESI-MS (Fig. 7f).73 For example, PAGE has been used to separate thiolated Au and Ag NCs with different sizes.74 The as-purified NC species were then ready for further ESI-MS measurement. In addition, PAGE can also be used to assess the purity of as-prepared metal NC samples.
3.3. Composition of thiolated Ag NCs
Composition is another important attribute of metal NCs. Besides mass spectrometry, energy-dispersive X-ray diffraction (EDX) and thermogravimetric analysis (TGA) can also be used to estimate the composition of metal NCs. In particular, each element has a unique atomic structure and shows a unique set of peaks in its X-ray spectrum, which makes EDX an efficient qualitative and quantitative analytical tool for elemental analysis of materials. EDX is usually attached to an electron microscope to analyze the composition of samples. Combined with the microscope, it can be used to analyze samples in a selected area, which may provide valuable information about the composition of metal NCs. TGA can also be used to estimate the ratio of Ag to thiolate ligands in the thiolated Ag NCs. The thiolate ligands on the surface of Ag NCs can be removed via high temperature treatment. The weight loss will then be used to determine the ratio of Ag to the organic ligand in the sample.
3.4. Structure of thiolated Ag NCs
In general, Ag NCs are composed of a number of Ag atoms and thiolate ligands, and feature some properties of supramolecules. One such property is a well-defined molecular structure. This property may not be seen in their larger counterparts – Ag NPs with core sizes above 2 nm. The cluster structure of Ag NCs, including the bonding between different constitutive atoms (particularly between the metal atoms and sulfur) and their spatial arrangement,88,101,112–117 is one crucial NC attribute, which can also be used to differentiate Ag NCs from their larger counterparts (Ag NPs). The NC structure also dictates the physical and chemical properties of thiolated metal NCs. Therefore, the determination of NC structure is one of the ultimate goals in the development of NC chemistry. The structural information of NCs can also shed some light on the fundamentals of the physicochemical properties of metal NCs, such as optical absorption, molecular chirality, and luminescence. It can also provide informative atomic-level understanding to correlate single metal atoms with large metal NPs (or nanocrystals). However, the resolving of metal NC structure is very challenging, and requires concerted efforts from both theoretical studies and combinative analytical techniques.
(1) Single crystal X-ray diffraction (XRD). XRD is used to determine the crystal structures of materials. In a particular XRD measurement, the set-up irradiates a sample with X-rays, and the diffraction angles are collected to determine the crystal structure. Recent studies show that some high-quality thiolated metal NCs (atomically precise) can be used to form single crystals (or supracrystals), typically through delicate control and design of the crystallization process.68,69 The high-quality NC single crystals can then be used to resolve the NC structure using single crystal XRD. Very few successful attempts have been recently reported on resolving the structures of thiolated Ag NCs. Further advances in this field require the community to specifically address the following three challenging issues. The first issue is the preparation of high-purity, atomically precise thiolated Ag NCs, in quantities large enough for optimization of the crystallization process. The formation of single crystals of thiolated Ag NCs has a strict requirement for the purity of the sample compound. Such efficient synthetic strategies are presently lacking, especially for those that are suitable for the synthesis of high-purity thiolated Ag NCs in the aqueous phase. The second issue is the relatively poorer stability of thiolated Ag NCs in solution (and sometimes in solid form) compared to thiolated Au NCs. The last challenging issue is the preparation of high-quality single crystals of thiolated Ag NCs. Reported procedures on the crystallization of metal NCs are still scarce. Therefore, more efforts are required to develop effective crystallization protocols for high-quality NC single crystals that are suitable for subsequent XRD measurement.
(2) Nuclear magnetic resonance (NMR). NMR is one of the most powerful techniques for determining the structures of organic molecules. It relies on the phenomenon of nuclear magnetic resonance and can provide detailed information about the structure and chemical environment of molecules. In particular, the intramolecular magnetic field around atoms in a molecule can change their resonance frequency, which can be used to provide detailed information on the electronic structures of the molecules. The NMR spectra of thiolated Ag NCs can provide informative data about the bonding between the Ag atoms and the surface ligands. For example, the NMR spectra of Ag32(SG)19 NC suggest its dominant motif to be [SR–Ag–SR] (Fig. 8a).87 In addition, two-dimensional (2D) NMR can also be used to illustrate the motif structures of thiolated Ag NCs. As shown in Fig. 8b and c, only one pair of peaks from CH3–CH2–S– was observed, with a ratio of 1
:
1 in intensity, which provided evidence for the equivalent environment of all the thiolate ligands on the Ag NC surface.
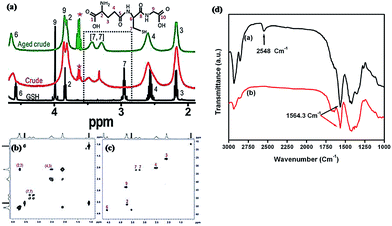 |
| Fig. 8 Structures of thiolated Ag NCs. (a) 1H NMR spectra of GSH (black curve) and Ag32(SG)19 NCs (red and green curves). (b) COSY and (c) HSQC spectra of Ag32(SG)19. (d) FTIR spectra of [a] dihydrolipoic acid (DHLA) and [b] DHLA-protected Ag NCs. Reprinted with permission.82,87 Copyright 2013, Royal Society of Chemistry. Copyright 2010, American Chemical Society. | |
(3) Fourier transform infrared (FTIR) spectroscopy. FTIR is a useful tool to study the surface chemistry of materials, as all functional groups feature a unique vibration and rotation, and possess a characteristic infrared absorption. Therefore, FTIR spectroscopy provides information about the functional groups on the metal NC surface. In addition, FTIR can also be used to investigate the structure of thiolated Ag NCs since the FTIR spectra of thiolate ligands may change upon their binding to the Ag core. For example, the disappearance of the characteristic peaks of S–H is often used to support the successful formation of thiolated Ag NCs, in which the deprotonated sulfur is bonded to Ag(I) on the NC surface (Fig. 8d).82
(4) X-ray photoelectron spectroscopy (XPS). XPS is a surface-sensitive spectroscopic technique that can be used to measure the elemental composition, the oxidation state of the metal core, and the bonding between the metal core and the protecting ligands. In a typical XPS measurement, monoenergetic soft X-rays are used to irradiate the NC samples, and the energies of the ejected electrons are measured. The XPS technique has been extensively used to verify the oxidation states of Au NCs. However, the same was not seen in the Ag NC system, since the binding energies of Ag(0) and Ag(I) are very close, with a difference of only ∼0.1 eV.
3.5. Toxicity of thiolated Ag NCs
Cytotoxicity is an important concern for the potential biomedical applications of Ag NCs, similar to some studies demonstrated for other types of functional nanomaterials.118–128 The toxicity issue also reflects the biological properties of Ag NCs, and this is therefore discussed in this section to provide a broad overview of chemical, physical, and biological properties of thiolated Ag NCs. However, information on the potential toxicity of thiolated Ag NCs is still lacking in the literature. Some recent studies have suggested that the cytotoxicity of Ag NCs is fairly low under certain operation conditions. In addition, the toxicity of thiolated Ag NCs on human cells may also be affected by the oxidation state of Ag atoms on the NC surface. One example is shown in Fig. 9.119 In this study, the as-prepared Ag0-rich NCs (labelled Ag0-R NCs) were shown to be more toxic to human neonatal foreskin fibroblast cells (BJ) than the Ag+-rich NCs (labelled Ag+-R NCs). One possible reason is that the Ag0-rich NCs could produce more reactive oxygen species (ROS) compared to the Ag+-rich NCs. In particular, the Ag0-rich NCs could be oxidized in lysosomes to form Ag+, which could help generate more ROS inside the cells.
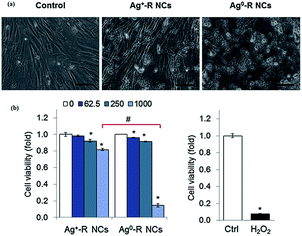 |
| Fig. 9 Cytotoxicity of GSH-protected Ag NCs with different oxidation states. Those cells treated with Ag+-R NCs showed a marginal cytotoxic effect when compared to Ag0-R NCs. Reprinted with permission.119 Copyright 2014, Elsevier. | |
4. Biomedical applications of thiolated Ag NCs
The ultrasmall thiolated Ag NCs feature unique physical, chemical, and biological properties, which make them good platforms to construct promising theranostic agents for biomedical applications. Some applications of thiolated Ag NCs have already surfaced in the community, and these are highlighted in this section.
4.1. Antimicrobial agents
In the last two decades, many studies have shown that Ag NPs are effective antimicrobial agents for a wide spectrum of microbes, although the working mechanism is still not very clear and this topic is presently under intense debate.129–133 There are several possible mechanisms for the effects of Ag NPs on microbes, including damage of the membrane, break up of the DNA, and the production of some active radicals, such as reactive oxygen species (ROS).134,135 From the materials perspective, Ag NCs are much smaller than Ag NPs, therefore, compared to NPs, Ag NCs may feature higher surface to volume ratios and more active surface atoms (with a unique electronic structure). As a result, one can expect higher antimicrobial efficacy for the ultrasmall Ag NCs as compared to their larger counterparts – Ag NPs.
For example, a recent study suggests that thiolated Ag NCs with core sizes of ∼1 nm performed better than large Ag NPs and traditional antibiotics to combat some bacteria, such as the multi-drug resistant bacteria. In particular, a GSH-protected Ag NC species showed higher efficacy against Escherichia coli (Gram negative) and Staphylococcus aureus (Gram positive) as compared to large Ag NPs with the same GSH coating.84 A similar observation was also valid for the case of multi-drug resistant bacteria, Pseudomonas aeruginosa (P. aeruginosa).60 As shown in Fig. 10a, the agar diffusion assay clearly suggests that 8 μg Ag16(SG)9 can effectively inhibit the growth of P. aeruginosa. The efficacy of thiolated Ag NCs was even better than that of the best antibiotics (e.g., chloramphenicol) against P. aeruginosa (Fig. 10b). The thiolated Ag NCs could kill the bacteria via generating a large number of intracellular ROS in the bacteria. The proposed mechanism was further confirmed by measuring the intracellular ROS in bacteria, showing a remarkable increase of ROS upon the introduction of Ag NCs (Fig. 10c). Another possible reason for the high antimicrobial activity of Ag NCs is their small particle size, which could facilitate the uptake of active Ag-NC-based antimicrobial agents by the bacteria. In addition, the oxidation state of Ag NCs also affects their antimicrobial activity.61
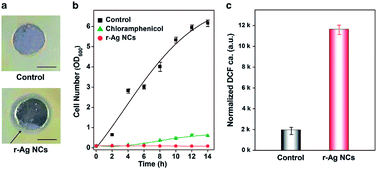 |
| Fig. 10 (a) Agar diffusion assay showing the presence of a clear zone surrounding the well where the red-emitting Ag NCs (labelled r-Ag NCs) were introduced (scale bar of 1 cm). (b) Comparison of cell numbers between the control, the sample with chloramphenicol, and the sample with r-Ag NCs within an incubation period of 14 h. (c) Comparison of the ROS concentration of cells treated with the control solution and the r-Ag NCs. Reprinted with permission.60 Copyright 2013, Nature Publishing Group. | |
4.2. Biosensing
Thiolated Ag NCs have recently emerged as promising luminescent probes for optical sensor development due to their strong luminescence and unique chemical properties. In addition, the size and surface chemistry of Ag NCs can be readily tailored to cater for sensor development for various biomolecules. For example, a recent study showed that a red-emitting Ag16(SG)9 NC was a good luminescent probe to detect a bio-thiol, cysteine (Cys), featuring high selectivity and sensitivity, as shown in Fig. 11.63 The sensor was constructed based upon the luminescence quenching of Ag16(SG)9 NCs by Cys molecules, where the Cys molecules could effectively break up the thiolated Ag NCs via the unique and strong Ag–thiolate interaction. However, the other 19 natural amino acids couldn’t do the same, since they don’t feature the thiol group. More interestingly, the as-constructed sensor could also differentiate Cys from other bio-thiols, such as glutathione (GSH). In this case, the steric hindrance of the protecting shell on the Ag NCs (GSH in this case) was readily used to differentiate small thiol molecules (Cys) from the bulky ones (GSH).
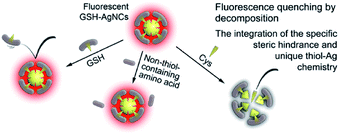 |
| Fig. 11 Ag16(SG)9 was used as a luminescent probe to construct an optical sensor that can selectively detect cysteine (Cys). Reprinted with permission.63 Copyright 2013, American Chemical Society. | |
4.3. Bioimaging
Similar to the biosensing applications, the strong luminescence of Ag NCs could also be used for bioimaging and biolabeling applications. For example, luminescent Ag NCs protected by GSH were used for the imaging of epithelial lung cancer cells (Fig. 12).93 The study suggests that the GSH-protected Ag NCs with red or blue emission could be easily detected by confocal microscopy under two-photon excitation. In addition, because of their small size, luminescent Ag NCs could also penetrate into the cells. Furthermore, the luminescent Ag NCs showed good photostability and colloidal stability in a wide pH range of 4 to 8. The strong luminescence and good stability of Ag NCs may make them good luminescent probes for bioimaging and biolabeling applications. It is also expected that the luminescence of thiolated Ag NCs could be further extended to the near-infrared region, which could further advance them for more biomedical applications in some particular biological settings.136
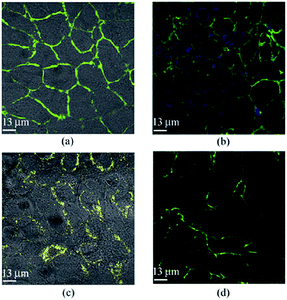 |
| Fig. 12 Fluorescence images of epithelial lung cancer cells (A549) after 4 h incubation with (a) GSH, (b) blue-emitting Ag NCs, (c) yellow-emitting Ag NCs, and (d) red-emitting Ag NCs. Reprinted with permission.91 Copyright 2012, Springer. | |
5. Conclusions
In the past decade, significant advances have been made in the field of thiolated Ag NCs, including their synthesis, characterization, and biomedical applications. For example, a number of efficient synthetic strategies have been developed to produce high-quality thiolated Ag NCs with well-defined size, structure, and surface. With delicate design of the synthetic chemistry, a bottom-up process can be readily used to prepare high-quality Ag NCs, either via a one-step or two-step protocol. In addition, some recent efforts have been devoted to understanding the formation process of thiolated Ag NCs. Concurrently with the recent advances in the synthesis of thiolated Ag NCs, some high-resolution analytical techniques have emerged as powerful tools to characterize thiolated Ag NCs, including (just to name a few) UV-vis absorption spectroscopy, PL spectroscopy, CD, TEM, PAGE, MALDI-TOF, ESI-MS, EDX, XPS, XRD, NMR, and FTIR. Such techniques have been regularly used in the laboratory to determine the physical and chemical properties of thiolated Ag NCs. They can also be useful to assess the purity of as-prepared Ag NC compounds. Moreover, the well-defined and well-characterized physicochemical properties of thiolated Ag NCs have motivated researchers to explore their applications in a wide spectrum of fields, such as biomedical, energy, environmental, and catalysis. The applications of thiolated Ag NCs in the biomedical field, such as serving as a novel type of antimicrobial agents or as promising luminescent probes for biosensing and bioimaging applications, have been demonstrated in the past few years, showing superior performance as compared to some other conventional materials.
However, although we have witnessed remarkable progress in thiolated Ag NCs in the past few years, some challenging issues still remain. Such issues may limit further developments of this field, and therefore require concerted efforts from the community. For example, efficient synthetic strategies for high-purity atomically precise Ag NCs are still lacking, especially synthetic strategies that are able to produce high-quality Ag NCs in the aqueous phase and also of quantities large enough for further fundamental and applied research. Therefore, more interesting and delicate synthetic chemistries are required to further facilitate the formation of thiolated Ag NCs with well-defined size, structure, and surface. In addition, further developments also call for efficient synthetic chemistries that could produce Ag NCs with the same core size, but protected by different types of thiolate ligands. Such materials are of interest to both basic and applied research, such as to study the ligand effects on the physical and chemical properties of Ag NCs, and to explore the practical applications where a delicately controlled NC surface could be crucial. It should also be noted that the development of efficient synthetic strategies is largely dependent on our understanding of the formation mechanism of thiolated Ag NCs, which is presently lacking in current studies.137 An in-depth understanding of the underlying chemistry and the formation mechanism of thiolated Ag NCs requires concerted efforts from both theorists and experimentalists, as has been somewhat demonstrated recently in the field of Au NCs.20,137–139 Some principles and techniques could be further adapted for the Ag system.
It has been recognized that the development of NC chemistry in the last two decades largely depended on the advances of high-resolution analytical techniques. Some emerging characterization techniques, such as ESI-MS and single crystal XRD, have already been used widely in determining the molecular-like properties of thiolated Ag NCs, including their molecular formulae and structures.68–70,73,74,140 Some key issues remain, especially for thiolated Ag NCs in the aqueous phase, featuring relatively poorer stability compared to those in the organic phase.44,60 It should also be noted that only very few thiolated Ag NCs (e.g., Ag44(MBA)30, Ag32(SG)19, Ag16(SG)9, Ag9(MSA)7, Ag8(MSA)8, Ag7(MSA)7, and Ag7(DMSA)4) have been identified with a precise cluster formula,60,68,71,73,90 many fewer than those in the Au NC system, where more than 20 thiolated Au NC species have been assigned with a precise cluster formula (e.g., Au10(SR)10,18,141,142 Au15(SR)13,17,18 Au18(SR)14,17,18,143 Au19(SR)13,144 Au20(SR)16,145 Au22(SR)18,22 Au23(SR)16,146 Au24(SR)20,147–149 Au25(SR)18,15,20,21,150,151 Au28(SR)20,34 Au29(SR)20,152 Au30(SR)18,153–155 Au36(SR)23,156 Au36(SR)24,11 Au38(SR)24,12,157–159 Au40(SR)24,160–162 Au41(SR)12,163 Au44(SR)28,164,165 Au55(SR)31,166 Au67(SR)35,167 Au68(SR)34,168 Au99(SR)42,169 Au102(SR)44,9,170 Au103–105(SR)45–46,171 Au130(SR)50,172–174 Au144(SR)60,175 Au187(SR)68).172 The same situation is valid for the cluster structure; only very few thiolated Ag NCs have been resolved with molecular structures (e.g., Ag44(MBA)30),68,69,176 many fewer than those in the Au system (e.g., Au20,177 Au23,146 Au24,148,149 Au25,10,150,178 Au28,34 Au30,154,155 Au36,11 Au38,12 and Au102).9 Such situations are expected to improve in the next few years with more efforts from researchers of multidisciplinary fields, including theorists and experimentalists working in cluster chemistry, noble metal chemistry, crystallography, etc.
While some studies have suggested that thiolated Ag NCs are promising for many applications, much more effort is needed to further pave their way towards practical applications. For the biomedical applications of thiolated Ag NCs, one important concern is the potential cytotoxicity of the functional Ag NCs, including their short-, middle-, and long-term effects. In addition, it will be of interest to establish the structure–property relationships of thiolated Ag NCs, which may further optimize their performance in a particular biological setting, as well as minimizing their biological implications. It should also be noted that a systematic and detailed understanding of the effects of functional Ag NCs in complex biological media, including cells, tissues, and animals, is presently lacking, therefore calling for more thorough and systematic studies in the near future, both in vitro and in vivo. This in-depth understanding may in turn provide useful design criteria for materials scientists to further engineer their materials for biomedical applications.
In summary, we hope this review article will further increase the interests of researchers in developing functional thiolated Ag NCs, from both the basic research, such as developing more efficient synthetic chemistries for high-quality thiolated Ag NCs, to develop more high-resolution analytical techniques for thorough and informative characterizations of as-prepared Ag NC compounds, or to further understand the fundamentals of chemical and physical properties and the formation mechanisms of thiolated Ag NCs; and the applied research, such as the development of a new type of antimicrobial agent for combating infectious diseases, and the design of promising luminescent probes for a wide spectrum of bioimaging and biosensing applications.
Acknowledgements
This work is financially supported by the Ministry of Education, Singapore, under the grants R-279-000-409-112 and R-279-000-383-112. K. Zheng acknowledges the National University of Singapore for her research scholarship.
Notes and references
- M. C. Daniel and D. Astruc, Chem. Rev., 2004, 104, 293–346 CrossRef CAS PubMed
. - P. K. Jain, K. S. Lee, I. H. El-Sayed and M. A. El-Sayed, J. Phys. Chem. B, 2006, 110, 7238–7248 CrossRef CAS PubMed
. - K. L. Kelly, E. Coronado, L. L. Zhao and G. C. Schatz, J. Phys. Chem. B, 2003, 107, 668–677 CrossRef CAS
. - E. C. Dreaden, A. M. Alkilany, X. Huang, C. J. Murphy and M. A. El-Sayed, Chem. Soc. Rev., 2012, 41, 2740–2779 RSC
. - R. Jin, Nanoscale, 2010, 2, 343–362 RSC
. - Q. Zhang, J. Xie, Y. Yu and J. Y. Lee, Nanoscale, 2010, 2, 1962–1975 RSC
. - Y. Lu and W. Chen, Chem. Soc. Rev., 2012, 41, 3594–3623 RSC
. - M. Zhu, C. M. Aikens, F. J. Hollander, G. C. Schatz and R. Jin, J. Am. Chem. Soc., 2008, 130, 5883–5885 CrossRef CAS PubMed
. - P. D. Jadzinsky, G. Calero, C. J. Ackerson, D. A. Bushnell and R. D. Kornberg, Science, 2007, 318, 430–433 CrossRef CAS PubMed
. - M. W. Heaven, A. Dass, P. S. White, K. M. Holt and R. W. Murray, J. Am. Chem. Soc., 2008, 130, 3754–3755 CrossRef CAS PubMed
. - C. Zeng, H. Qian, T. Li, G. Li, N. L. Rosi, B. Yoon, R. N. Barnett, R. L. Whetten, U. Landman and R. Jin, Angew. Chem., Int. Ed., 2012, 51, 13114–13118 CrossRef CAS PubMed
. - H. Qian, W. T. Eckenhoff, Y. Zhu, T. Pintauer and R. Jin, J. Am. Chem. Soc., 2010, 132, 8280–8281 CrossRef CAS PubMed
. - H. Qian, M. Zhu, Z. Wu and R. Jin, Acc. Chem. Res., 2012, 45, 1470–1479 CrossRef CAS PubMed
. - J. Akola, M. Walter, R. L. Whetten, H. Hakkinen and H. Gronbeck, J. Am. Chem. Soc., 2008, 130, 3756–3757 CrossRef CAS PubMed
. - X. Yuan, B. Zhang, Z. Luo, Q. Yao, D. T. Leong, N. Yan and J. Xie, Angew. Chem., Int. Ed., 2014, 53, 4623–4627 CrossRef CAS PubMed
. - X. Dou, X. Yuan, Q. Yao, Z. Luo, K. Zheng and J. Xie, Chem. Commun., 2014, 50, 7459–7462 RSC
. - Q. Yao, Y. Yu, X. Yuan, Y. Yu, J. Xie and J. Y. Lee, Small, 2013, 9, 2696–2701 CrossRef CAS PubMed
. - Y. Yu, X. Chen, Q. Yao, Y. Yu, N. Yan and J. Xie, Chem. Mater., 2013, 25, 946–952 CrossRef CAS
. - Y. Yu, Z. Luo, C. S. Teo, Y. N. Tan and J. Xie, Chem. Commun., 2013, 49, 9740–9742 RSC
. - Y. Yu, Z. T. Luo, Y. Yu, J. Y. Lee and J. P. Xie, ACS Nano, 2012, 6, 7920–7927 CrossRef CAS PubMed
. - X. Yuan, Y. Yu, Q. Yao, Q. Zhang and J. Xie, J. Phys. Chem. Lett., 2012, 3, 2310–2314 CrossRef CAS
. - Y. Yu, Z. Luo, D. M. Chevrier, D. T. Leong, P. Zhang, D. E. Jiang and J. Xie, J. Am. Chem. Soc., 2014, 136, 1246–1249 CrossRef CAS PubMed
. - R. W. Murray, Chem. Rev., 2008, 108, 2688–2720 CrossRef CAS PubMed
. - S. Chen, R. S. Ingram, M. J. Hostetler, J. J. Pietron, R. W. Murray, T. G. Schaaff, J. T. Khoury, M. M. Alvarez and R. L. Whetten, Science, 1998, 280, 2098–2101 CrossRef CAS
. - S. Antonello, G. Arrigoni, T. Dainese, M. De Nardi, G. Parisio, L. Perotti, A. René, A. Venzo and F. Maran, ACS Nano, 2014, 8, 2788–2795 CrossRef CAS PubMed
. - O. Varnavski, G. Ramakrishna, J. Kim, D. Lee and T. Goodson, J. Am. Chem. Soc., 2010, 132, 16–17 CrossRef CAS PubMed
. - G. Ramakrishna, O. Varnavski, J. Kim, D. Lee and T. Goodson, J. Am. Chem. Soc., 2008, 130, 5032–5033 CrossRef CAS PubMed
. - H. C. Weissker, H. B. Escobar, V. D. Thanthirige, K. Kwak, D. Lee, G. Ramakrishna, R. L. Whetten and X. Lopez-Lozano, Nat. Commun., 2014, 5, 3785 Search PubMed
. - M. Walter, J. Akola, O. Lopez-Acevedo, P. D. Jadzinsky, G. Calero, C. J. Ackerson, R. L. Whetten, H. Gronbeck and H. Hakkinen, Proc. Natl. Acad. Sci. U. S. A., 2008, 105, 9157–9162 CrossRef CAS PubMed
. - E. B. Guidez and C. M. Aikens, Nanoscale, 2012, 4, 4190–4198 RSC
. - Y. Negishi, H. Tsunoyama, M. Suzuki, N. Kawamura, M. M. Matsushita, K. Maruyama, T. Sugawara, T. Yokoyama and T. Tsukuda, J. Am. Chem. Soc., 2006, 128, 12034–12035 CrossRef CAS PubMed
. - M. Zhu, C. M. Aikens, M. P. Hendrich, R. Gupta, H. Qian, G. C. Schatz and R. Jin, J. Am. Chem. Soc., 2009, 131, 2490–2492 CrossRef CAS PubMed
. - S. Antonello, N. V. Perera, M. Ruzzi, J. A. Gascon and F. Maran, J. Am. Chem. Soc., 2013, 135, 15585–15594 CrossRef CAS PubMed
. - C. Zeng, T. Li, A. Das, N. L. Rosi and R. Jin, J. Am. Chem. Soc., 2013, 135, 10011–10013 CrossRef CAS PubMed
. - M. Zhu, H. Qian, X. Meng, S. Jin, Z. Wu and R. Jin, Nano Lett., 2011, 11, 3963–3969 CrossRef CAS PubMed
. - S. Knoppe, O. A. Wong, S. Malola, H. Häkkinen, T. Bürgi, T. Verbiest and C. J. Ackerson, J. Am. Chem. Soc., 2014, 136, 4129–4132 CrossRef CAS PubMed
. - L. Shang, S. J. Dong and G. U. Nienhaus, Nano Today, 2011, 6, 401–418 CrossRef CAS PubMed
. - I. Diez and R. H. Ras, Nanoscale, 2011, 3, 1963–1970 RSC
. - S. Choi, R. M. Dickson and J. Yu, Chem. Soc. Rev., 2012, 41, 1867–1891 RSC
. - J. Zheng, C. Zhang and R. M. Dickson, Phys. Rev. Lett., 2004, 93, 077402 CrossRef
. - J. Xie, Y. Zheng and J. Y. Ying, J. Am. Chem. Soc., 2009, 131, 888–889 CrossRef CAS PubMed
. - J. Yu, S. A. Patel and R. M. Dickson, Angew. Chem., Int. Ed., 2007, 46, 2028–2030 CrossRef CAS PubMed
. - Z. Luo, X. Yuan, Y. Yu, Q. Zhang, D. T. Leong, J. Y. Lee and J. Xie, J. Am. Chem. Soc., 2012, 134, 16662–16670 CrossRef CAS PubMed
. - X. Yuan, Z. Luo, Q. Zhang, X. Zhang, Y. Zheng, J. Y. Lee and J. Xie, ACS Nano, 2011, 5, 8800–8808 CrossRef CAS PubMed
. - J. Zheng, C. Zhou, M. Yu and J. Liu, Nanoscale, 2012, 4, 4073–4083 RSC
. - L. C. Ho, C. W. Wang, P. Roy and H. T. Chang, J. Chin. Chem. Soc., 2014, 61, 163–174 CrossRef CAS
. - P. Wu, K. Hwang, T. Lan and Y. Lu, J. Am. Chem. Soc., 2013, 135, 5254–5257 CrossRef CAS PubMed
. - D. Y. Chen, Z. T. Luo, N. J. Li, J. Y. Lee, J. P. Xie and J. M. Lu, Adv. Funct. Mater., 2013, 23, 4324–4331 CrossRef CAS
. - X. Yuan, Q. Yao, Y. Yu, Z. Luo, X. Dou and J. Xie, J. Phys. Chem. Lett., 2013, 4, 1811–1815 CrossRef CAS
. - C. V. Conroy, J. Jiang, C. Zhang, T. Ahuja, Z. Tang, C. A. Prickett, J. J. Yang and G. Wang, Nanoscale, 2014, 6, 7416–7423 RSC
. - Z. Tang, T. Ahuja, S. Wang and G. Wang, Nanoscale, 2012, 4, 4119–4124 RSC
. - S. Choi, R. M. Dickson, J.-K. Lee and J. Yu, Photochem. Photobiol. Sci., 2012, 11, 274–278 CAS
. - S. Choi, S. Park, K. Lee and J. Yu, Chem. Commun., 2013, 49, 10908–10910 RSC
. - P.-C. Chen, J.-Y. Ma, L.-Y. Chen, G.-L. Lin, C.-C. Shih, T.-Y. Lin and H.-T. Chang, Nanoscale, 2014, 6, 3503–3507 RSC
. - Z. Yuan, Y.-C. Chen, H.-W. Li and H.-T. Chang, Chem. Commun., 2014, 50, 9800–9815 RSC
. - Y.-C. Shiang, C.-C. Huang, W.-Y. Chen, P.-C. Chen and H.-T. Chang, J. Mater. Chem., 2012, 22, 12972–12982 RSC
. - J. Sharma, R. C. Rocha, M. L. Phipps, H.-C. Yeh, K. A. Balatsky, D. M. Vu, A. P. Shreve, J. H. Werner and J. S. Martinez, Nanoscale, 2012, 4, 4107–4110 RSC
. - J. S. Mohanty, P. L. Xavier, K. Chaudhari, M. Bootharaju, N. Goswami, S. Pal and T. Pradeep, Nanoscale, 2012, 4, 4255–4262 RSC
. - H. Xu and K. S. Suslick, Adv. Mater., 2010, 22, 1078–1082 CrossRef CAS PubMed
. - X. Yuan, M. I. Setyawati, A. S. Tan, C. N. Ong, D. T. Leong and J. Xie, NPG Asia Mater., 2013, 5, e39 CrossRef CAS
. - X. Yuan, M. I. Setyawati, D. T. Leong and J. Xie, Nano Res., 2014, 7, 301–307 CrossRef CAS PubMed
. - X. Yuan, Z. Luo, Y. Yu, Q. Yao and J. Xie, Chem.–Asian J., 2013, 8, 858–871 CrossRef CAS PubMed
. - X. Yuan, Y. Tay, X. Dou, Z. Luo, D. T. Leong and J. Xie, Anal. Chem., 2013, 85, 1913–1919 CrossRef CAS PubMed
. - X. Yuan, T. J. Yeow, Q. Zhang, J. Y. Lee and J. Xie, Nanoscale, 2012, 4, 1968–1971 RSC
. - Y. Cui, Y. Wang, R. Liu, Z. Sun, Y. Wei, Y. Zhao and X. Gao, ACS Nano, 2011, 5, 8684–8689 CrossRef CAS PubMed
. - H. C. Yeh, J. Sharma, I. M. Shih, D. M. Vu, J. S. Martinez and J. H. Werner, J. Am. Chem. Soc., 2012, 134, 11550–11558 CrossRef CAS PubMed
. - J. T. Petty, S. P. Story, J. C. Hsiang and R. M. Dickson, J. Phys. Chem. Lett., 2013, 4, 1148–1155 CrossRef CAS PubMed
. - A. Desireddy, B. E. Conn, J. Guo, B. Yoon, R. N. Barnett, B. M. Monahan, K. Kirschbaum, W. P. Griffith, R. L. Whetten, U. Landman and T. P. Bigioni, Nature, 2013, 501, 399–402 CrossRef CAS PubMed
. - H. Yang, Y. Wang, H. Huang, L. Gell, L. Lehtovaara, S. Malola, H. Hakkinen and N. Zheng, Nat. Commun., 2013, 4, 2422 Search PubMed
. - Z. Wu, E. Lanni, W. Chen, M. E. Bier, D. Ly and R. Jin, J. Am. Chem. Soc., 2009, 131, 16672–16674 CrossRef CAS PubMed
. - T. U. B. Rao, B. Nataraju and T. Pradeep, J. Am. Chem. Soc., 2010, 132, 16304–16307 CrossRef CAS PubMed
. - L. G. AbdulHalim, S. Ashraf, K. Katsiev, A. R. Kirmani, N. Kothalawala, D. H. Anjum, S. Abbas, A. Amassian, F. Stellacci and A. Dass, J. Mater. Chem. A, 2013, 1, 10148–10154 CAS
. - J. Guo, S. Kumar, M. Bolan, A. Desireddy, T. P. Bigioni and W. P. Griffith, Anal. Chem., 2012, 84, 5304–5308 CrossRef CAS PubMed
. - S. Kumar, M. D. Bolan and T. P. Bigioni, J. Am. Chem. Soc., 2010, 132, 13141–13143 CrossRef CAS PubMed
. - Z. Luo, K. Zheng and J. Xie, Chem. Commun., 2014, 50, 5143–5155 RSC
. - Y. Yu, Q. Yao, Z. Luo, X. Yuan, J. Y. Lee and J. Xie, Nanoscale, 2013, 5, 4606–4620 RSC
. - N. Goswami, K. Zheng and J. Xie, Nanoscale, 2014, 6, 13328–13347 RSC
. - M. R. Branham, A. D. Douglas, A. J. Mills, J. B. Tracy, P. S. White and R. W. Murray, Langmuir, 2006, 22, 11376–11383 CrossRef CAS PubMed
. - Y. Negishi, R. Arai, Y. Niihori and T. Tsukuda, Chem. Commun., 2011, 47, 5693–5695 RSC
. - N. Cathcart, P. Mistry, C. Makra, B. Pietrobon, N. Coombs, M. Jelokhani-Niaraki and V. Kitaev, Langmuir, 2009, 25, 5840–5846 CrossRef CAS PubMed
. - M. A. Muhammed, F. Aldeek, G. Palui, L. Trapiella-Alfonso and H. Mattoussi, ACS Nano, 2012, 6, 8950–8961 CrossRef CAS PubMed
. - B. Adhikari and A. Banerjee, Chem. Mater., 2010, 22, 4364–4371 CrossRef CAS
. - I. Chakraborty, T. Udayabhaskararao and T. Pradeep, Chem. Commun., 2012, 48, 6788–6790 RSC
. - I. Chakraborty, T. Udayabhaskararao, G. K. Deepesh and T. Pradeep, J. Mater. Chem. B, 2013, 1, 4059–4064 RSC
. - T. Zhou, M. Rong, Z. Cai, C. J. Yang and X. Chen, Nanoscale, 2012, 4, 4103–4106 RSC
. - B. S. González, M. Blanco and M. A. López-Quintela, Nanoscale, 2012, 4, 7632–7635 RSC
. - T. Udayabhaskararao, M. Bootharaju and T. Pradeep, Nanoscale, 2013, 5, 9404–9411 RSC
. - I. Chakraborty, A. Govindarajan, J. Erusappan, A. Ghosh, T. Pradeep, B. Yoon, R. L. Whetten and U. Landman, Nano Lett., 2012, 12, 5861–5866 CrossRef CAS PubMed
. - I. Chakraborty, T. Udayabhaskararao and T. Pradeep, J. Hazard. Mater., 2012, 211–212, 396–403 CrossRef CAS PubMed
. - T. Udaya Bhaskara Rao and T. Pradeep, Angew. Chem., Int. Ed., 2010, 49, 3925–3929 CrossRef CAS PubMed
. - K. V. Mrudula, T. U. B. Rao and T. Pradeep, J. Mater. Chem., 2009, 19, 4335–4342 RSC
. - L. Dhanalakshmi, T. Udayabhaskararao and T. Pradeep, Chem. Commun., 2012, 48, 859–861 RSC
. - X. Le Guével, C. Spies, N. Daum, G. Jung and M. Schneider, Nano Res., 2012, 5, 379–387 CrossRef PubMed
. - I. Chakraborty, J. Erusappan, A. Govindarajan, K. S. Sugi, T. Udayabhaskararao, A. Ghosh and T. Pradeep, Nanoscale, 2014, 6, 8024–8031 RSC
. - N. Nishida, H. Yao, T. Ueda, A. Sasaki and K. Kimura, Chem. Mater., 2007, 19, 2831–2841 CrossRef CAS
. - R. Jiang, B. Li, C. Fang and J. Wang, Adv. Mater., 2014, 26, 5274–5309 CrossRef CAS PubMed
. - L. M. Liz-Marzán, C. J. Murphy and J. Wang, Chem. Soc. Rev., 2014, 43, 3820–3822 RSC
. - Q. B. Zhang, Y. N. Tan, J. P. Xie and J. Y. Lee, Plasmonics, 2009, 4, 9–22 CrossRef CAS
. - J. Huang, Y. Zhu, M. Lin, Q. Wang, L. Zhao, Y. Yang, K. X. Yao and Y. Han, J. Am. Chem. Soc., 2013, 135, 8552–8561 CrossRef CAS PubMed
. - M. Pelton, Y. Tang, O. M. Bakr and F. Stellacci, J. Am. Chem. Soc., 2012, 134, 11856–11859 CrossRef CAS PubMed
. - O. M. Bakr, V. Amendola, C. M. Aikens, W. Wenseleers, R. Li, L. Dal Negro, G. C. Schatz and F. Stellacci, Angew. Chem., Int. Ed., 2009, 48, 5921–5926 CrossRef CAS PubMed
. - K. S. Sugi, I. Chakraborty, T. Udayabhaskararao, J. S. Mohanty and T. Pradeep, Part. Part. Syst. Charact., 2013, 30, 241–243 CrossRef CAS
. - M. Farrag, M. Thamer, M. Tschurl, T. Burgi and U. Heiz, J. Phys. Chem. C, 2012, 116, 8034–8043 CAS
. - T. H. Lee and R. M. Dickson, Proc. Natl. Acad. Sci. U. S. A., 2003, 100, 3043–3046 CrossRef CAS PubMed
. - C. M. Aikens, S. Li and G. C. Schatz, J. Phys. Chem. C, 2008, 112, 11272–11279 CAS
. - G.-T. Bae and C. M. Aikens, J. Phys. Chem. C, 2012, 116, 10356–10367 CAS
. - N. Nishida, H. Yao and K. Kimura, Langmuir, 2008, 24, 2759–2766 CrossRef CAS PubMed
. - M. Farrag, M. Tschurl and U. Heiz, Chem. Mater., 2013, 25, 862–870 CrossRef CAS
. - N. Cathcart and V. Kitaev, J. Phys. Chem. C, 2010, 114, 16010–16017 CAS
. - Y. Zhu, J. He, C. Shang, X. Miao, J. Huang, Z. Liu, H. Chen and Y. Han, J. Am. Chem. Soc., 2014, 136, 12746–12752 CrossRef CAS PubMed
. - M. Azubel, J. Koivisto, S. Malola, D. Bushnell, G. L. Hura, A. L. Koh, H. Tsunoyama, T. Tsukuda, M. Pettersson, H. Hakkinen and R. D. Kornberg, Science, 2014, 345, 909–912 CrossRef CAS PubMed
. - H. Yang, J. Lei, B. Wu, Y. Wang, M. Zhou, A. Xia, L. Zheng and N. Zheng, Chem. Commun., 2013, 49, 300–302 RSC
. - H. Y. Yang, Y. Wang and N. F. Zheng, Nanoscale, 2013, 5, 2674–2677 RSC
. - H. Xiang, S. H. Wei and X. Gong, J. Am. Chem. Soc., 2010, 132, 7355–7360 CrossRef CAS PubMed
. - Y. Sun, K. Balasubramanian, T. U. B. Rao and T. Pradeep, J. Phys. Chem. C, 2011, 115, 20380–20387 CAS
. - B. Bellina, I. Compagnon, F. Bertorelle, M. Broyer, R. Antoine, P. Dugourd, L. Gell, A. Kulesza, R. Mitrić and V. Bonačić-Koutecký, J. Phys. Chem. C, 2011, 115, 24549–24554 CAS
. - F. Bertorelle, R. Hamouda, D. Rayane, M. Broyer, R. Antoine, P. Dugourd, L. Gell, A. Kulesza, R. Mitric and V. Bonacic-Koutecky, Nanoscale, 2013, 5, 5637–5643 RSC
. - M. Giovanni, C. Y. Tay, M. I. Setyawati, J. Xie, C. N. Ong, R. Fan, J. Yue, L. Zhang and D. T. Leong, Environ. Toxicol., 2014 DOI:10.1002/tox.22015
. - C. Y. Tay, W. Fang, M. I. Setyawati, S. L. Chia, K. S. Tan, C. H. L. Hong and D. T. Leong, ACS Appl. Mater. Interfaces, 2014, 6, 6248–6256 CAS
. - M. I. Setyawati, C. Y. Tay and D. T. Leong, Nanomedicine, 2014, 9, 369–371 CrossRef CAS PubMed
. - C. Y. Tay, P. Cai, M. I. Setyawati, W. Fang, L. P. Tan, C. H. Hong, X. Chen and D. T. Leong, Nano Lett., 2014, 14, 83–88 CrossRef CAS PubMed
. - M. I. Setyawati, C. Y. Tay and D. T. Leong, Biomaterials, 2013, 34, 10133–10142 CrossRef CAS PubMed
. - C. Y. Tay, W. R. Fang, M. I. Setyawati, C. P. Sum, J. P. Xie, K. W. Ng, X. D. Chen, C. H. L. Hong and D. T. Leong, Part. Part. Syst. Charact., 2013, 30, 784–793 CrossRef CAS
. - M. I. Setyawati, C. Y. Tay, S. L. Chia, S. L. Goh, W. Fang, M. J. Neo, H. C. Chong, S. M. Tan, S. C. Loo, K. W. Ng, J. P. Xie, C. N. Ong, N. S. Tan and D. T. Leong, Nat. Commun., 2013, 4, 1673 CrossRef CAS PubMed
. - Y. Zhao, J. L. Howe, Z. Yu, D. T. Leong, J. J. Chu, J. S. Loo and K. W. Ng, Small, 2013, 9, 387–392 CrossRef PubMed
. - M. I. Setyawati, W. Fang, S. L. Chia and D. T. Leong, Asia-Pac. J. Chem. Eng., 2013, 8, 205–217 CrossRef CAS
. - X. Ma, L. H. Zhang, L. R. Wang, X. Xue, J. H. Sun, Y. Wu, G. Zou, X. Wu, P. C. Wang, W. G. Wamer, J. J. Yin, K. Zheng and X. J. Liang, ACS Nano, 2012, 6, 10486–10496 CAS
. - X. W. Ma, Y. Y. Wu, S. B. Jin, Y. Tian, X. N. Zhang, Y. L. Zhao, L. Yu and X. J. Liang, ACS Nano, 2011, 5, 8629–8639 CrossRef CAS PubMed
. - V. K. Sharma, R. A. Yngard and Y. Lin, Adv. Colloid Interface Sci., 2009, 145, 83–96 CrossRef CAS PubMed
. - J. S. Kim, E. Kuk, K. N. Yu, J. H. Kim, S. J. Park, H. J. Lee, S. H. Kim, Y. K. Park, Y. H. Park, C. Y. Hwang, Y. K. Kim, Y. S. Lee, D. H. Jeong and M. H. Cho, Nanomed.: Nanotechnol., Biol. Med., 2007, 3, 95–101 CrossRef CAS PubMed
. - I. Sondi and B. Salopek-Sondi, J. Colloid Interface Sci., 2004, 275, 177–182 CrossRef CAS PubMed
. - S. Pal, Y. K. Tak and J. M. Song, Appl. Environ. Microbiol., 2007, 73, 1712–1720 CrossRef CAS PubMed
. - J. R. Morones, J. L. Elechiguerra, A. Camacho, K. Holt, J. B. Kouri, J. T. Ramirez and M. J. Yacaman, Nanotechnology, 2005, 16, 2346–2353 CrossRef CAS PubMed
. - Q. Li, S. Mahendra, D. Y. Lyon, L. Brunet, M. V. Liga, D. Li and P. J. Alvarez, Water Res., 2008, 42, 4591–4602 CrossRef CAS PubMed
. - Q. L. Feng, J. Wu, G. Q. Chen, F. Z. Cui, T. N. Kim and J. O. Kim, J. Biomed. Mater. Res., 2000, 52, 662–668 CrossRef CAS
. - S. P. Gao, D. H. Chen, Q. W. Li, J. Ye, H. Jiang, C. Amatore and X. M. Wang, Sci. Rep., 2014, 4, 4384 Search PubMed
. - Z. Luo, V. Nachammai, B. Zhang, N. Yan, D. T. Leong, D. E. Jiang and J. Xie, J. Am. Chem. Soc., 2014, 136, 10577–10580 CrossRef CAS PubMed
. - R. C. Jin, H. F. Qian, Z. K. Wu, Y. Zhu, M. Z. Zhu, A. Mohanty and N. Garg, J. Phys. Chem. Lett., 2010, 1, 2903–2910 CrossRef CAS
. - Y. Shichibu, Y. Negishi, H. Tsunoyama, M. Kanehara, T. Teranishi and T. Tsukuda, Small, 2007, 3, 835–839 CrossRef CAS PubMed
. - K. M. Harkness, Y. Tang, A. Dass, J. Pan, N. Kothalawala, V. J. Reddy, D. E. Cliffel, B. Demeler, F. Stellacci, O. M. Bakr and J. A. McLean, Nanoscale, 2012, 4, 4269–4274 RSC
. - Y. Negishi, K. Nobusada and T. Tsukuda, J. Am. Chem. Soc., 2005, 127, 5261–5270 CrossRef CAS PubMed
. - X. D. Zhang, Z. Luo, J. Chen, X. Shen, S. Song, Y. Sun, S. Fan, F. Fan, D. T. Leong and J. Xie, Adv. Mater., 2014, 26, 4565–4568 CrossRef CAS PubMed
. - A. Ghosh, T. Udayabhaskararao and T. Pradeep, J. Phys. Chem. Lett., 2012, 3, 1997–2002 CrossRef CAS
. - Z. Wu, M. A. MacDonald, J. Chen, P. Zhang and R. Jin, J. Am. Chem. Soc., 2011, 133, 9670–9673 CrossRef CAS PubMed
. - M. Zhu, H. Qian and R. Jin, J. Am. Chem. Soc., 2009, 131, 7220–7221 CrossRef CAS PubMed
. - A. Das, T. Li, K. Nobusada, C. Zeng, N. L. Rosi and R. Jin, J. Am. Chem. Soc., 2013, 135, 18264–18267 CrossRef CAS PubMed
. - M. Zhu, H. Qian and R. Jin, J. Phys. Chem. Lett., 2010, 1, 1003–1007 CrossRef CAS
. - A. Das, T. Li, G. Li, K. Nobusada, C. Zeng, N. L. Rosi and R. Jin, Nanoscale, 2014, 6, 6458–6462 RSC
. - Y. Song, S. Wang, J. Zhang, X. Kang, S. Chen, P. Li, H. Sheng and M. Zhu, J. Am. Chem. Soc., 2014, 136, 2963–2965 CrossRef CAS PubMed
. - M. Zhu, E. Lanni, N. Garg, M. E. Bier and R. Jin, J. Am. Chem. Soc., 2008, 130, 1138–1139 CrossRef CAS PubMed
. - Y. Shichibu, Y. Negishi, T. Tsukuda and T. Teranishi, J. Am. Chem. Soc., 2005, 127, 13464–13465 CrossRef CAS PubMed
. - Y. Yu, Q. Yao, K. Cheng, X. Yuan, Z. Luo and J. Xie, Part. Part. Syst. Charact., 2014, 31, 652–656 CrossRef CAS
. - D. Crasto and A. Dass, J. Phys. Chem. C, 2013, 117, 22094–22097 CAS
. - D. Crasto, S. Malola, G. Brosofsky, A. Dass and H. Hakkinen, J. Am. Chem. Soc., 2014, 136, 5000–5005 CrossRef CAS PubMed
. - H. Yang, Y. Wang, A. J. Edwards, J. Yan and N. Zheng, Chem. Commun., 2014, 50, 14325–14327 RSC
. - P. R. Nimmala and A. Dass, J. Am. Chem. Soc., 2011, 133, 9175–9177 CrossRef CAS PubMed
. - H. Qian, Y. Zhu and R. Jin, ACS Nano, 2009, 3, 3795–3803 CrossRef CAS PubMed
. - N. K. Chaki, Y. Negishi, H. Tsunoyama, Y. Shichibu and T. Tsukuda, J. Am. Chem. Soc., 2008, 130, 8608–8610 CrossRef CAS PubMed
. - L. Beqa, D. Deschamps, S. Perrio, A.-C. Gaumont, S. Knoppe and T. Bürgi, J. Phys. Chem. C, 2013, 117, 21619–21625 CAS
. - S. Malola, L. Lehtovaara, S. Knoppe, K. J. Hu, R. E. Palmer, T. Burgi and H. Hakkinen, J. Am. Chem. Soc., 2012, 134, 19560–19563 CrossRef CAS PubMed
. - S. Knoppe, I. Dolamic, A. Dass and T. Burgi, Angew. Chem., Int. Ed., 2012, 51, 7589–7591 CrossRef CAS PubMed
. - H. Qian, Y. Zhu and R. Jin, J. Am. Chem. Soc., 2010, 132, 4583–4585 CrossRef CAS PubMed
. - J. Nishigaki, R. Tsunoyama, H. Tsunoyama, N. Ichikuni, S. Yamazoe, Y. Negishi, M. Ito, T. Matsuo, K. Tamao and T. Tsukuda, J. Am. Chem. Soc., 2012, 134, 14295–14297 CrossRef CAS PubMed
. - C. Zeng, Y. Chen, G. Li and R. Jin, Chem. Commun., 2014, 50, 55–57 RSC
. - Y. Pei, S. Lin, J. Su and C. Liu, J. Am. Chem. Soc., 2013, 135, 19060–19063 CrossRef CAS PubMed
. - H. Qian and R. Jin, Chem. Commun., 2011, 47, 11462–11464 RSC
. - P. R. Nimmala, B. Yoon, R. L. Whetten, U. Landman and A. Dass, J. Phys. Chem. A, 2013, 117, 504–517 CrossRef CAS PubMed
. - A. Dass, J. Am. Chem. Soc., 2009, 131, 11666–11667 CrossRef CAS PubMed
. - G. Li, C. Zeng and R. Jin, J. Am. Chem. Soc., 2014, 136, 3673–3679 CrossRef CAS PubMed
. - Y. Levi-Kalisman, P. D. Jadzinsky, N. Kalisman, H. Tsunoyama, T. Tsukuda, D. A. Bushnell and R. D. Kornberg, J. Am. Chem. Soc., 2011, 133, 2976–2982 CrossRef CAS PubMed
. - A. Dass, P. R. Nimmala, V. R. Jupally and N. Kothalawala, Nanoscale, 2013, 5, 12082–12085 RSC
. - Y. Negishi, C. Sakamoto, T. Ohyama and T. Tsukuda, J. Phys. Chem. Lett., 2012, 3, 1624–1628 CrossRef CAS
. - V. R. Jupally and A. Dass, Phys. Chem. Chem. Phys., 2014, 16, 10473–10479 RSC
. - A. Tlahuice-Flores, U. Santiago, D. Bahena, E. Vinogradova, C. V. Conroy, T. Ahuja, S. B. Bach, A. Ponce, G. Wang, M. Jose-Yacaman and R. L. Whetten, J. Phys. Chem. A, 2013, 117, 10470–10476 CrossRef CAS PubMed
. - H. Qian and R. Jin, Chem. Mater., 2011, 23, 2209–2217 CrossRef CAS
. - B. Yoon, W. D. Luedtke, R. N. Barnett, J. Gao, A. Desireddy, B. E. Conn, T. Bigioni and U. Landman, Nat. Mater., 2014, 13, 807–811 CrossRef CAS PubMed
. - C. Zeng, C. Liu, Y. Chen, N. L. Rosi and R. Jin, J. Am. Chem. Soc., 2014, 136, 11922–11925 CrossRef CAS PubMed
. - T. Dainese, S. Antonello, J. A. Gascón, F. Pan, N. V. Perera, M. Ruzzi, A. Venzo, A. Zoleo, K. Rissanen and F. Maran, ACS Nano, 2014, 8, 3904–3912 CrossRef CAS PubMed
.
|
This journal is © The Royal Society of Chemistry 2014 |