DOI:
10.1039/C4RA06702A
(Paper)
RSC Adv., 2014,
4, 49953-49959
Antiproliferative and apoptosis-induction studies of a metallosurfactant in human breast cancer cell MCF-7
Received
6th July 2014
, Accepted 22nd September 2014
First published on 22nd September 2014
Abstract
The cytotoxic potential of the surfactant–cobalt(III) complex (metallosurfactant) cis-[Co(trien)(C14H29NH2)Cl](ClO4)2 was tested on the MCF-7 breast cancer cell. The viability of the treated cell was evaluated by adopting MTT assay. The mode of cell death was assessed by adopting different morphological, cellular and molecular methods such as comet assay for DNA damage and apoptosis assays (Hoechst staining, acridine orange & ethidium bromide (AO & EB) staining and Annexin V-Cy3 assay). Mitochondria-mediated apoptosis was tested using JC-1 dye. Cell cycle analysis was made by adopting flow cytometry, and the expression of some key pro- and anti-apoptotic proteins was analyzed by adopting Western blotting. The surfactant–cobalt(III) complex induced cell death in a dose- and time-dependent manner. The mode of cell death was essentially apoptosis though necrosis was also noticed. Flow cytometric analysis indicated that the treatment caused cell cycle arrest, as indicated in the accumulation of cells in the sub-G0 + G1 compartment. Western blot analysis revealed the up-regulation of pro-apoptotic p53 and Bax proteins and down-regulation of anti-apoptotic Bcl-2 protein. The study revealed the antiproliferative and apoptosis-induction properties of the surfactant–cobalt(III) complex in an MCF-7 breast cancer cell, primarily by inducing DNA damage and possibly through elevation of ROS levels.
Introduction
Numerous drug delivery and drug targeting systems have been studied in an attempt to minimize drug degradation and loss to prevent harmful side effects and to increase the drug's bioavailability at the biological target.1,2 Surfactant systems, in particular, can solubilize poorly soluble drugs (with a low hydrophilicity), and thereby increase their bioavailability. Moreover, they may allow the drug to stay in the body for an enough long period to provide a gradual accumulation at the required site and lead to a build-up of the pharmacological agent.3,4
Metallosurfactant (i.e., surfactant–metal complex) is a special type of surfactant in which a coordination complex (containing a central metal ion surrounded by ligands coordinated to the metal) acts as the surfactant (Fig. 1). In metallosurfactants, the entity containing the central metal ion along with its primary coordination sphere acts as the head group, and the hydrophobic entity of one or more of the ligands acts as the tail part. Similar to any other well-known surfactant, for example sodium dodecyl sulfate (SDS), these metallosurfactants also form micelles in an aqueous solution above a specific concentration called a critical micelle concentration (cmc). Metallosurfactants have received a sustained high level of attention from the scientific community for the past few years due to their relevance in various redox processes taking place in biological systems, and they could be promising agents for biological activities such as anti-cancer, anti-candida and anti-microbial.5–7
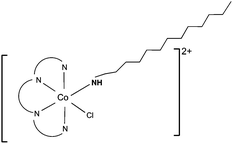 |
| Fig. 1 Structure of the surfactant–cobalt(III) complex, cis-[Co(trien)(C14H29NH2)Cl](ClO4)2 (trien = triethylenetetramine, C14H29NH2 = tetradecylamine). | |
Studies show that variations in length, degree of unsaturation, flexibility, and chemical structure of the lipid chain as well as in the nature of the counter ion can have a considerable effect on the transfection efficiency of the surfactant system.8 In fact, a change or replacement of the surfactant by another amphiphile with different molecular structural features may induce high transfection effectiveness.9 Hence, elucidation of the optimal transfection recipe for any given cell line appears to require a prior accurate knowledge of the surfactant. We have been interested in the synthesis and micelle forming properties of cobalt(III) complexes containing lipophilic ligands.10,11 We have previously described our results pertaining to the interaction of some surfactant–cobalt(III) complexes with DNA and serum albumins.11–13 We were interested in finding if such an interaction can be of use in dealing with cancer cells. Herein, we report the antiproliferative and apoptosis-inducing properties of one of these surfactant–cobalt(III) complexes (Fig. 1), which has been already shown to bind calf thymus DNA and possess antibiotic property.12
There are two forms of cell death, namely apoptosis and necrosis. Necrotic death results from collapse and disturbance of the ionic balance in cells due to toxic insult. It is characterized by swelling of cellular organelles and loss of plasma membrane integrity, impairing the cell's ability to produce ATP. Apoptosis, on the other hand, is programmed cell death, which commonly occurs in cells which are meant to be removed without affecting the neighboring normal cells.14 Apoptosis is characterized by chromatin marginalization, nuclear fragmentation, and apoptotic body formation. This sequence of changes depends on the ability of the dying cell to engage in ATP-dependent processes of self-degradation. Recent studies have demonstrated that in response to a given death stimulus there is often a continuum of apoptosis and necrosis. Many toxic insults induce apoptosis at lower doses and necrosis at higher doses. Even in response to a certain dose of a death-inducing agent, features of both apoptosis and necrosis may coexist in the same cell. In addition, if not engulfed by neighboring cells or in cell culture, where phagocytosis does not usually happen, cells in the late stages of apoptosis may present necrotic features due to loss of cellular energy and plasma membrane integrity. This process is called “apoptotic necrosis”, “necroptosis” or “secondary necrosis”.15 The response of the cell to a DNA damaging agent, which can be apoptosis, necrosis or necroptosis, is important in understanding the application of the agent in cancer therapy. Thus, in this study we targeted the mode of cell death in an MCF-7 breast cancer cell when treated with the chosen surfactant–cobalt(III) complex.
Experimental
Materials
The procedure for the synthesis of the cis-[Co(trien)(C14H29NH2)Cl](ClO4)2 of the present study is reported in our earlier work.12 The human breast cancer cell MCF-7 was obtained from National Centre for Cell Science (NCCS), Pune, India.
Methods
Cell culture. MCF-7 breast cancer cells were cultured as a monolayer in RPMI-1640 medium (Biochrom AG, Berlin, Germany), supplemented with 10% fetal bovine serum (Sigma-Aldrich, St. Louis, MO, USA) and with 100 U mL−1 penicillin and 100 μg mL−1 streptomycin as antibiotics (Himedia, Mumbai, India), at 37 °C in a humidified atmosphere of 5% CO2 in a CO2 incubator (Heraeus, Hanau, Germany).
MTT assay16. The surfactant–cobalt(III) complex was dissolved in dimethyl sulfoxide (DMSO). The cells were seeded in 96-well plates at a density of 5 × 103 cells per well and treated with the complex at concentrations ranging from 5 to 50 μM for 24 and 48 h with DMSO as the solvent control. At the end of the exposure period, the cells were incubated with 20 μL 3-(4,5-di-methylthiazol-2-yl)-2,5-diphenyl-2H-tetrazolium bromide (MTT), and the plates were wrapped with aluminum foil and incubated for another 4 h. Then, the culture medium was aspirated, and 100 μL of DMSO was added to dissolve the formazan product. The absorbance was monitored at 570 nm (measurement) and 630 nm (reference) using a microplate reader (Bio-Rad, Hercules, CA, USA). Data were collected for four replicates each and used to calculate the respective means and the standard deviations. The percentage inhibition was calculated from this data using the formula:
% inhibition = ((mean OD of untreated cells (control) − mean OD of treated cells)/mean OD of untreated cells (control)) × 100. |
The IC50 value was determined as the concentration of the complex that is required to reduce the absorbance to half that of the control.
Acridine orange (AO) and ethidium bromide (EB) staining17. MCF-7 cells were seeded in 6-well plates and allowed to reach 70% confluence. The cells were then treated with the IC50 concentration of the complex for 24 and 48 h. The cells were trypsinized and pelleted, and then suspended in PBS. A drop of cell suspension was placed on a glass slide and stained with AO & EB, and a cover slip was laid over to reduce light diffraction. At random, 300 cells were observed in a fluorescent microscope (Carl Zeiss, Jena, Germany) fitted with a 377–355 nm filter, and observed at 400× magnification. The percentage of cells reflecting pathological changes was calculated. Data were collected for four replicates each and used to calculate the respective means and the standard deviations.
Hoechst 33258 staining18. The cell suspension, as mentioned above, was stained with Hoechst 33258 and incubated at 37 °C for 15 min. At random, 300 cells were observed in the fluorescent microscope at 400× magnification, and the percentage of cells reflecting pathological changes was calculated. Data were collected for four replicates each and used to calculate the respective means and the standard deviations.
Single-cell gel electrophoresis assay. DNA damage in individual cells was quantified by adopting comet assay.19 The cells were treated with the surfactant–cobalt(III) complex for 24 and 48 h. The harvested cells were suspended in low-melting-point agarose in PBS and pipetted out to microscope slides pre-coated with a layer of normal-melting-point agarose. Slides were chilled on ice for 10 min, and then immersed in lysis solution (2.5 M NaCl, 100 mM Na2EDTA, 10 mM Tris, 0.2 mM NaOH, pH 10.01 and Triton X-100), and the solution was kept overnight at 4 °C in order to lyse the cells and to permit DNA unfolding. Thereafter, the slides were exposed to alkaline buffer (300 mM NaOH, 1 mM Na2EDTA, pH > 13) for 20 min to allow DNA unwinding. The slides were washed with buffer (0.4 M Tris, pH 7.5) to neutralize excess alkali and to remove detergents before staining with ethidium bromide (20 μL, 50 μg mL−1). Photomicrographs were obtained using the fluorescent microscope. One hundred and fifty cells from each treatment group were digitalized and analyzed using CASP software. The images were used to determine the DNA content of individual nuclei and to evaluate the degree of DNA damage represented by the fraction of total DNA in the tail.
Measurement of mitochondrial transmembrane potential (ΔΨm)18. Mitochondrial transmembrane potential, ΔΨm, is an important parameter of mitochondrial function used as an indicator of cell health. JC-1 is a lipophilic, cationic dye that can selectively enter the mitochondria and reversibly change color from green to red as the membrane potential increases. The cells were grown on glass cover slips placed in 6-well plates and treated with the IC50 concentration of the surfactant–cobalt(III) complex. The cells were stained with JC-1 dye after 24 and 48 h exposure. The mitochondrial depolarization patterns of the cells were observed in the fluorescent microscope at 400× magnification.
Annexin V-Cy3 staining. Phosphatidylserine translocation from the inner to the outer leaflet of the plasma membrane is one of the early features of apoptosis. Cell surface phosphatidylserine was detected using phosphatidylserine-binding protein annexin V conjugated with Cy3 using the commercially available annexin V-Cy3 apoptosis detection kit (APOAC, Apoptosis Detection Kit, Sigma). Cells were cultured and treated with the IC50 concentration of the complex and incubated for 24 and 48 h. The cells were processed according to the instructions in the kit and observed in the fluorescent microscope. The combination of 6-carboxyfluorescein diacetate (6-CFDA) with annexin V conjugated to Cy3 in the kit allowed the differentiation of live cells (green), necrotic cells (red) and apoptotic cells (red and green). At random, 300 cells were observed, and the percentage of cells reflecting cell death (both apoptotic and necrotic) was calculated. Data were collected in two separate experiments, each in quadruplicate, and used to calculate the respective means and the standard deviations.
Cell cycle analysis. The cells were seeded in 25 cm2 culture flasks at a density of 1 × 106 cells per flask. After 24 h, the cells were treated with IC50 concentration of the complex and incubated for 24 and 48 h. The cells were trypsinized, harvested and fixed in 1 mL 80% cold ethanol in test tubes and incubated at 4 °C for 15 min. After incubation, cells were centrifuged at 1500 rpm for 5 min, and the cell pellets were re-suspended in 500 μL propidium iodide (PI) (10 μg mL−1) containing 300 μg mL−1 RNase (Sigma-Aldrich, St. Louis, MO, USA). The cells were then incubated on ice for 30 min and filtered through 53 μm nylon mesh. Cell cycle distribution was analyzed using FACScan (Becton–Dickinson, Sanjose, CA) with 15 mW, 488 nm argon ion laser. PI signals were collected using 585/42-band pass filter. The data were acquired and analyzed with Cell Quest software.
Western blot analysis. For Western blotting, cells were treated with the complex at IC50 concentration for 12 and 24 h, and appropriate amounts of cell lysates (25 μg protein) were resolved over 10% Tris–glycine polyacrylamide gel, and then transferred onto the PVDF membrane. The blots were blocked using 5% non-fat dry milk and probed using p53, Bcl-2 and Bax primary monoclonal antibodies in blocking buffer overnight at 4 °C. The membrane was then incubated with appropriate secondary antibody-horseradish peroxidase conjugate (Amersham Life Sciences Inc., IL, USA), followed by detection using chemiluminescence ECL kit (Amersham Life Sciences Inc., IL, USA). To ensure equal loading of protein, the membrane was stripped and reprobed with anti-β-actin antibody (Sigma-Aldrich, USA).
Results
MTT assay. To evaluate the cytotoxic activity of the surfactant–cobalt(III) complex on human breast cancer cells (MCF-7), the cells were treated with semilog doses of the complex. After 24 and 48 h, cell viability was determined by adopting MTT assay. The complex was cytotoxic to cells in a dose- and duration-dependent manner. Dose- and duration-dependence curves were obtained for determining the IC50 for this complex. The IC50 of the complex for MCF-7 cell were 12 ± 2 μM for 24 h and 8 ± 2 μM for 48 h treatment. The time- and dose-dependent decrease in cell viability indicated that the surfactant–cobalt(III) complex enters the cells slowly and kills the cells gradually.
Hoechst 33258 staining. After the cells were treated with the respective IC50 concentrations of the complex for 24 and 48 h, the cells were observed for cytological changes by adopting Hoechst 33258 staining. The observations revealed that the treatment with the complex brought about marginalization and/or fragmentation of chromatin, bi-nucleation, cytoplasmic vacuolation, nuclear shrinkage, cytoplasmic blebbing and late apoptosis indications of dot-like chromatin (Fig. 2A). These cytological changes strongly indicated that the cells were committed to cell death, more by apoptosis than necrosis, in a dose- as well as duration-dependent manner. The data collected from the counting of cells with normal and abnormal nuclear features are shown in the Fig. 2B.
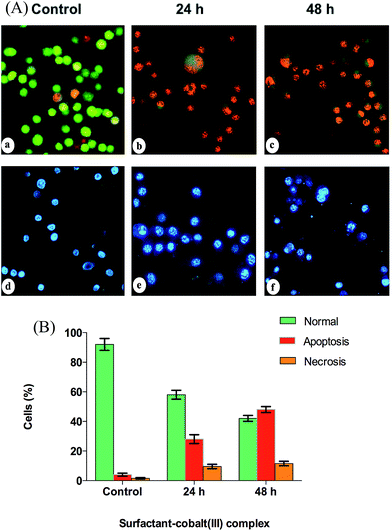 |
| Fig. 2 (A) Photographs showing the feature of AO/EB (a–c) & Hoechst 33528 (d–f) stained MCF-7 breast cancer cells. Control and treated with cis-[Co(trien)(C14H29NH2)Cl](ClO4)2 for 24 h and 48 h. (B) Bar diagram of percent normal, apoptotic and necrotic cells as revealed by AO & EB staining. | |
Acridine orange and ethidium bromide (AO & EB) staining. AO & EB staining followed by fluorescence microscopy revealed apoptosis from the perspective of fluorescence emission. The cells were classified into four types according to the fluorescence emission and the morphological features of chromatin condensation in the nuclei. (1) The viable cells had uniformly green fluorescing nuclei with a highly organized structure. (2) Early apoptotic cells (which possessed intact membrane but DNA fragmentation was initiated) had green fluorescing nuclei, but peri-nuclear chromatin condensation was visible as bright green patches or fragments. (3) Late apoptotic cells had orange to red fluorescing nuclei with condensed or fragmented chromatin. (4) Necrotic cells had uniformly orange to red fluorescing nuclei with no indication of chromatin fragmentation, and the cells were swollen. Data collected from counting of cells with the four different fluorescence patterns are shown in Fig. 2. The surfactant–cobalt(III) complex-treated MCF-7 cells showed higher incidence of apoptosis than necrosis, i.e., about 35% apoptosis and 10% necrosis in 24 h treatment and 55% apoptosis and 12% necrosis in 48 h treatment (Fig. 2). In the 48 h treatment group, the percentage of apoptotic cells was higher than normal cells, which indicates that the incidence of apoptosis increased in duration-dependent manner but the ratio of necrosis between 24 and 48 h treatments did not show a significant difference.
DNA damage. As shown in Fig. 3, the comet images were used to determine the DNA content of individual nuclei and to evaluate the degree of DNA damage representing the fraction of total DNA in the tail. The cells were classified into five groups: 0–20% (intact), 20–40% (slightly damaged), 40–60% (damaged), 60–80% (highly damaged) and >80% (dead). The surfactant–cobalt(III) complex induced DNA damage in MCF-7 cells at 24 h and 48 h. As shown in Fig. 3, the surfactant–cobalt(III) complex-treated cells reflected DNA damage, which increased when the cells were exposed for a longer duration.
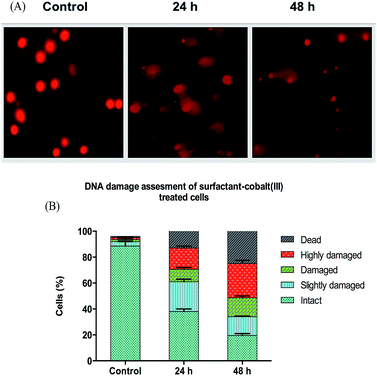 |
| Fig. 3 (A) DNA fragmentation in the surfactant–cobalt(III) complex-treated MCF-7 breast cancer cells as revealed in the comet assay. Comet images of DNA double strand breaks at 24 h and 48 h treatment of the complex. The duration-dependence of the DNA damage is revealed. DNA damage in MCF-7 breast cancer cell (24 h, 48 h) populations as defined according to the percentage of DNA in the tail. (B) The multiple parts of each column represent (from bottom to top) intact (0–20%), slightly damaged (20–40%), damaged (40–60%), highly damaged (60–80%), and dead (80–100%). | |
Alteration in mitochondrial transmembrane potential (ΔΨm). Early cellular apoptosis is always accompanied with disruption of mitochondrial membrane ΔΨm, resulting in rapid collapse of the electrochemical gradient. The disruption of ΔΨm by the complex was examined using a mitochondrion-specific dye, JC-1. In this study, the control cells showed red fluorescence due to an intact mitochondrial transmembrane potential. The surfactant–cobalt(III) complex-treated cells showed progressive loss of red JC-aggregate fluorescence and appearance of cytoplasmic diffusion of green monomer fluorescence at 24 h and complete loss of red fluorescence and presence of only green fluorescence at 48 h (Fig. 4).
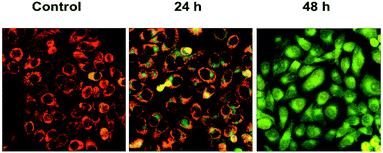 |
| Fig. 4 Photomicrographs of transmembrane potential-dependent JC-1 staining of mitochondria in control and treated MCF-7 breast cancer cells. In untreated cells, high mitochondrial polarization is indicated by red fluorescence due to JC-aggregate formation by the concentrated dye. In treated cells, depolarized regions are indicated by the green fluorescence of JC-1 monomers. | |
Translocation of phosphatidylserine. An early indicator of apoptosis is the rapid translocation and accumulation of the membrane phospholipid, phosphatidylserine, from the cytoplasmic interface to the extracellular surface. This loss of membrane symmetry can be detected by utilizing the binding properties of annexin. The live untreated cells were stained only with 6-CFDA and they emitted green fluorescence. The cells treated with the surfactant–cobalt(III) complex and stained with Annexin V-Cy3 showed an increase in the number of cells double-stained with annexin V-Cy3 and 6-CFDA (red and green fluorescence), indicating early apoptosis. The number of cells that were late apoptotic and necrotic (red emission) increased at 48 h treatment (Fig. 5).
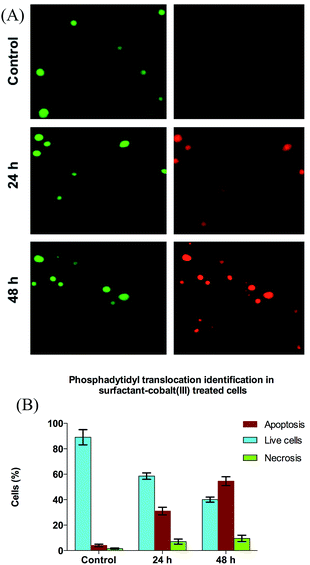 |
| Fig. 5 (A) MCF-7 breast cancer cells stained with Annexin V-Cy3. Cells were treated with the surfactant–cobalt(III) complex for 24 and 48 h. (B) Bar diagram of percent live, necrotic and apoptotic cells as revealed by Annexin V-Cy3 assay followed by manual counting. | |
Cell cycle arrest. The DNA content of 10
000 cells of each sample was evaluated, and the overall results revealed duration-dependent distribution of treated cells different from the respective controls. As shown in Fig. 6, there was a significant increase in cells in the sub-G0 + G1 compartment and decrease in cells in G0 + G1 and S phases, indicating that the treatment of cells with the surfactant–cobalt(III) complex for 24 and 48 h inhibited the progression of cells from sub-G0 + G1 to S phase, further indicating that the surfactant–cobalt(III) complex caused an impairment of cell cycle progression.
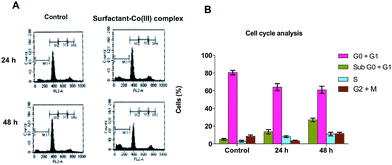 |
| Fig. 6 (A) Data showing antiproliferative potential of surfactant–cobalt(III) complex on MCF-7 cancer cells. Cell cycle arrest was caused by the surfactant–cobalt(III) complex treatment, as detected by flow cytometry. (B) Percentages of S, G1, G2 cell cycle phases and of apoptotic cells, determined by sub-G1 peak quantitation, in surfactant–cobalt(III) complex-treated MCF-7 cells. | |
Expression levels of p53, Bcl-2 and Bax. We analyzed the expression of p53 (tumor suppressor protein) and two Bcl-2 family members, Bax and Bcl-2, in the surfactant–cobalt(III) complex-treated MCF-7 cells adopting Western blotting. Compared with the untreated cells, we found up-regulation of p53 protein in the surfactant–cobalt(III) complex-treated cells. Moreover, the treatment significantly down-regulated Bcl-2 protein and up-regulated Bax protein in MCF-7 cells in the treatment group (Fig. 7). Overall, the results suggested a late onset of necrosis due to alteration in the mitochondrial transmembrane potential. The change in the expression of these proteins clearly indicated that the complex might be triggering ROS machinery such as to lead to apoptosis.
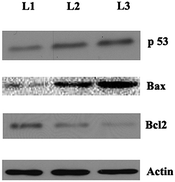 |
| Fig. 7 Western blot of p53, Bax and Bcl-2 proteins in MCF-7 cells. Lane 1 (L1) – MCF-7 cells (control); lanes 2 (L2) and 3 (L3) – cells treated with the surfactant–cobalt(III) complex at 12 h (L2) and for 24 h (L3). | |
Discussion
Medicinal inorganic chemistry can potentially exploit the unique properties of metal ions for the design of new drugs. This has, for instance, led to the clinical application of platinum-based agents for cancer treatment, such as cisplatin, carboplatin and oxaliplatin. However, the platinum drugs in current practice encounter many obstacles such as drug resistance and systemic toxicity in clinical applications.20 This has spurred chemists to adopt different strategies in the development of new metal-based anticancer agents with different mechanisms of action. These include analogues of cisplatin or oxaliplatin, monofunctional platinum(II) complexes, polynuclear platinum(II) complexes, trans-platinum(II) complexes, and platinum(IV) complexes.20 There are also attempts to find avenues for more selective delivery and/or activation of cisplatin-related pro-drugs21 and to discover new non-covalent interactions with the classical target, DNA.21 The use of the metal as scaffold rather than reactive center and the departure from the cisplatin paradigm of activity towards a more targeted, cancer cell-specific approach is a major trend in recent times.21
The outcome of MTT assay in this study is a clear indication that the surfactant–cobalt(III) complex inflicts cytotoxic effect in MCF-7 breast cancer cells and affects their viability when treated at even very low concentrations with the extent of damage depending upon the dose and the duration of treatment. It is suggested that the coordinated structure of the complex ligand might be playing a major role in time-dependent increase in the cytotoxicity of the complex. The hydrophobic forces in DNA interaction and the surfactant properties6,13 characteristic of the surfactant–cobalt(III) complex tested herein would have led to the cytotoxic effect.
The cytological changes observed by adopting AO & EB and Hoechst 33258 staining reveal that the complex brings about death overtly by apoptosis, which is indicated in the marginalization and/or fragmentation of chromatin, bi-/multi-nucleation, cytoplasmic vacuolation, cytoplasmic blebbing, late apoptosis indication of dot-like chromatin and apoptotic body formation. AO & EB staining combined with fluorescence microscopy revealed apoptosis from the perspective of fluorescence emission as well, wherein a fairly high percentage of treated cells was suggestive of death by apoptosis, though necrosis was also indicated more during the 48 h time point. The observations made from the assay for phosphatidylserine translocation from the inner to the outer leaflet of the plasma membrane (annexin V-Cy 3 assay), which is considered as one of the earliest events in apoptosis,22 further substantiates that apoptosis is the principal mode of cell death in the present case. Since the assay using JC-1 dye indicated a loss of mitochondrial transmembrane potential, the surfactant–cobalt(III) complex-induced apoptosis might have a link with high levels of ROS.23
Strand breaks in DNA were indicated in the comet assay, and this DNA damage caused by the surfactant–cobalt(III) treatment could be the principal basis for the cells becoming unviable and most of them taken to death by apoptosis.24 The complex tested in this study is capable of altering the nucleo-cytoplasmic environment by binding to DNA through a series of weak interactions such as hydrogen bonding, van der Waals interactions of functionalities bound along the grooves of the DNA helix and hydrophobic effects.12 Such an interaction within cells could thus be stress-mediated. DNA strand breaks are produced when anticancer drugs such as cisplatin and tenoposide induce oxidative stress via generation of ROS.25,26 Involvement of ROS in the surfactant–cobalt(III)-induced DNA damage is further reflected in some of the cells succumbing to necrotic death, particularly during 48 h treatment.
The surfactant–cobalt(III) complex treatment not only inhibited the MCF-7 cancer cell growth to significant levels, but it prevented their progression from G2/M of one cycle to G1 of the next cycle, and consequently there was duration-dependent accumulation of cells in sub-G0–G1 fractions, as revealed in the cell cycle analysis. This sub-G1 peak is widely regarded for representing hypo-diploid cells and is generally considered as an indicator of apoptotic cell death.27 The presence of a sub-G1 peak demonstrated that apoptosis occurred mostly in the G1 phase, although we cannot exclude, based on the present cytometric evidence, that apoptotic death could also take place in other cell cycle phases. Taken together, these data open interesting perspectives in the study of the relationship between induction of DNA damage and commitment to apoptosis in response to the surfactant–cobalt(III) complex treatment.28,29 Thus, the duration-dependent accumulation of cells in the sub-G0–G1 fraction strongly suggests that the mode of action of the complex might be dependent on the complex-specificity, and its interactions could be associated with DNA damage.
The p53 is a tumor suppressor protein, which can function as a transcription factor. It controls cell proliferation and apoptosis in response to various types of cellular stresses or damage.30 It is known that in most human cancer cells, the loss of functional p53 impairs the response of cells to apoptotic stimuli. Apoptosis triggered by p53 has been reported to be dependent on an increase of ROS and on the release of pro-apoptotic factors resulting from mitochondrial damage.31 The mitochondrial membrane-based Bcl-2 oncoprotein and other related proteins may play an important role in determining whether cells undergo apoptosis. Increased expression of Bax can induce apoptosis by suppressing the activity of Bcl-2 (ref. 32–34). It was also reported that the ratio of Bcl-2 to Bax, rather than Bcl-2 alone, is important for drug-induced apoptosis.35 In our study, the up-regulation of p53 began to increase during the early hours of treatment, and then decreased gradually. The comparison of results between apoptotic response and induction of p53 indicated that the up-regulation of p53 caused induction of apoptosis during the early hours. On the other hand, the surfactant–cobalt(III) complex treatment significantly down-regulated Bcl-2 protein and elevated the levels of Bax protein in MCF-7 cells. Overall, the results suggest that as a consequence of the events induced by the complex, there is alteration in the mitochondrial membrane-bound protein expression and p53.
In general, DNA damage, phosphatidylserine externalization, loss of mitochondrial trans-membrane potential and strong hypodiploid accumulation of cells treated with the surfactant–cobalt(III) complex lead to the inhibition of cell cycle progression and death of cells, primarily by apoptosis. The surfactant–cobalt(III) complex appears to be capable of killing p53-positive breast cancer cells. However, it would be pertinent to analyze the mechanisms of cell death in the present context by finding the expression of pro- and anti-apoptotic genes and caspases in the case of apoptosis and oxidative damage in the case of necrosis.
Conclusions
In conclusion, we have demonstrated that the surfactant–cobalt(III) complex inhibits proliferation and induces cell death significantly by apoptosis and to some extent by necrosis in the p53-positive breast cancer cell. At the molecular level, this complex reflected an up-regulation of pro-apoptotic proteins p53 and Bax and down-regulation of anti-apoptotic protein Bcl-2. Thus, the surfactant–cobalt(III) complex provided a strong evidence of induction of apoptosis, which could be of application in cancer therapy. The necrotic death of cells, occurring under certain circumstances and due to high levels of ROS, also is a desired end point in cancer therapy.
Acknowledgements
MAA is grateful to the Doerenkamp-Zbinden Foundation (DZF), Switzerland, and King Saud University, Kingdom of Saudi Arabia, for the financial support. RA thanks Council of Scientific and Industrial Research (CSIR), Government of India, New Delhi, for the Senior Research Fellowship (CSIR/09/475(0163)/2010-EMR-1). MZ acknowledges the Maulana Azad National Fellowship of University Grants Commisiion (UGC), New Delhi (F1-17.1/2011/MANF-MUS-WES-7390/(SA-III/Website)).
Notes and references
- N. Martinho, C. Damge and C. P. Reis, J. Biomater. Nanobiotechnol., 2011, 2, 510 CrossRef CAS.
- B. V. Bonifácio, P. B. Silva, M. A. S. Ramos, K. M. S. Negri, T. M. Bauab and M. Chorilli, Int. J. Nanomed., 2014, 9, 1 CrossRef PubMed.
- K. Kawakami, N. Oda, K. Miyoshi, T. Funaki and Y. Ida, Eur. J. Pharm. Sci., 2006, 28, 1 CrossRef PubMed.
- C. O. Rangei-Yagui, A. Pessoa Junior and C. Tavares, J. Pharm. Pharm. Sci., 2005, 8, 147 Search PubMed.
- A. M. Badwai, M. A. Mohamed, M. Z. Mohamed and M. M. Khowdairy, J. Cancer Res. Ther., 2007, 3, 198 CrossRef PubMed.
- A. M. Badawi, N. I. Zakhary, S. M. I. Morsy, G. M. Sabry, M. M. Fouad and A. M. Mousa, Am. J. Sci., 2012, 8, 763 Search PubMed.
- A. Ismail, M. H. M. Ahmed, A. Hessein and M. Ali, J. Dispersion Sci. Technol., 2011, 33, 1144 Search PubMed.
- H. Wang, T. Kaur, N. Tavakoli, J. Joseph and S. Wettig, Phys. Chem. Chem. Phys., 2013, 15, 20510 RSC.
- R. Leventis and J. R. Silvius, Biochim. Biophys. Acta., 1990, 1023, 124 CrossRef CAS.
- K. Sasikala and S. Arunachalam, Monatsh. Chem., 2010, 141, 309 CrossRef CAS.
- R. Senthilkumar, S. Arunachalam, V. S. Periasamy, C. P. Preethy, A. Riyasdeen and M. A. Akbarsha, J. Inorg. Biochem., 2009, 103, 117 CrossRef PubMed.
- R. Senthilkumar and S. Arunachalam, Biophys. Chem., 2008, 136, 136 CrossRef PubMed.
- R. Senthilkumar, P. Paul, A. Riyasdeen, G. Wagniéres, H. van den Bergh, M. A. Akbarsha and S. Arunachalam, Colloids Surf., B, 2011, 86, 35 CrossRef PubMed.
- F. R. Kerr, A. H. Wyllie and A. R. Currie, Br. J. Cancer, 1972, 26, 239 CrossRef.
- G. Majno and I. Joris, Am. J. Pathol., 1995, 146, 3 CAS.
- T. Mosmann, J. Immunol. Methods, 1983, 65, 55 CrossRef CAS.
- D. L. Spector, R. D. Goldman and L. A. Leinwand, Cell: A Laboratory Manual, Culture and Biochemical Analysis of Cells, Cold Spring Harbor Laboratory Press, New York, 1998 Search PubMed.
- G. P. Kasibhatla, D. Finucane, T. Brunner, E. B. Wetzel and D. R. Green, Cell: a laboratory manual culture and biochemical analysis of cells, CSHL Press, 2000 Search PubMed.
- N. P. Singh, M. T. McCoy, R. R. Tice and E. L. Schneider, Exp. Cell Res., 1988, 175, 184 CrossRef CAS.
- X. Wang, Anti-Cancer Agents Med. Chem., 2010, 10, 396 CrossRef CAS.
- P. C. Bruijnincx and P. J. Sadler, Curr. Opin. Chem. Biol., 2008, 12, 197 CrossRef CAS PubMed.
- E. Vermes, C. Haanen, H. Steffens-Nakken and C. Reutellingsperger, J. Immunol. Methods, 1995, 184, 39 CrossRef.
- S. H. Inayat-Hussain, B. O. Annuar, L. B. Din, A. M. Ali and D. Ross, Toxicol. In Vitro, 2003, 17, 433 CrossRef CAS.
- S. Yasuhara, Y. Zhu, T. Matsui, N. Tipirneni, Y. Yasuhara, M. Kaneki, A. Rosenzweig and J. A. Martyn, J. Histochem. Cytochem., 2003, 51, 873 CrossRef CAS PubMed.
- J. Fukutomi, A. Fukuda, S. Fukuda, M. Hara, A. Terada and M. Yoshida, Life Sci., 2006, 80, 254 CrossRef CAS PubMed.
- A. M. Havelka, M. Berndtsson, M. H. Olofsson, M. C. Shoshan and S. Linder, Mini-Rev. Med. Chem., 2007, 7, 1035 CrossRef CAS.
- A. Choi, J. Y. Kim, J. Y. Lee, C. M. Kang, H. J. Kwon, Y. D. Yoo, T. W. Kim, Y. S. Lee and S. J. Lee, Int. J. Oncol., 2001, 19, 837 Search PubMed.
- Y. Mao, K. J. Liu, J. J. Jiang and X. Shi, J. Toxicol. Environ. Health, 1996, 47, 61 CrossRef CAS PubMed.
- R. Mangiarotti, M. Danova, R. Alberici and C. Pellicciari, Br. J. Cancer, 1998, 77, 186 CrossRef CAS.
- T. Riley, E. Sontag, P. Chen and A. Levine, Nat. Rev. Mol. Cell Biol., 2008, 9, 402 CrossRef CAS PubMed.
- S. Matoba, J. G. Kang, W. D. Patino, A. Wragg, M. Boehm, O. Gavrilova, P. J. Hurley, F. Bunz and P. M. Hwang, Science, 2006, 312, 1650 CrossRef CAS PubMed.
- H. Boise, M. G. Garcia, C. E. Postema, L. Ding, T. Lindsten, L. A. Turks, X. Mao, G. Nuñez and C. B. Thompson, Cell, 1993, 74, 597 CrossRef.
- S. J. Korsmeyer, X. M. Yin, Z. N. Oltvai, D. J. Veis-Novack and G. P. Linette, Biochim. Biophys. Acta, 1995, 1271, 63 CrossRef.
- S. J. Korsmeyer, Cancer Res., 1999, 59, 1693 Search PubMed.
- L. Zhang, J. Yu, B. H. Park, K. W. Kinzler and B. Vogelstein, Science, 2000, 290, 989 CrossRef CAS.
|
This journal is © The Royal Society of Chemistry 2014 |