DOI:
10.1039/C3RA43011A
(Review Article)
RSC Adv., 2013,
3, 24734-24757
Biobutanol: the outlook of an academic and industrialist
Received
16th June 2013
, Accepted 5th September 2013
First published on 9th September 2013
Abstract
The gradual shift of transportation fuels from oil based fuels to alternative fuel resources and worldwide demand for energy has been the impetus for research to produce alcohol biofuels from renewable resources. Currently bioethanol and biodiesel can, however, not cover an increasing demand for biofuels. Hence, there is an extensive need for advanced biofuels with superior fuel properties. The present review is focused on the development of biobutanol, which is regarded to be superior to bioethanol in terms of energy density and hygroscopicity. Although acetone–butanol–ethanol (ABE) fermentation is one of the oldest large-scale fermentation processes, butanol yield by anaerobic fermentation remains sub-optimal. For sustainable industrial scale butanol production, a number of obstacles need to be addressed including choice of feedstock, low product yield, product toxicity to production strain, multiple end-products and downstream processing of alcohol mixtures. Metabolic engineering provides a means for fermentation improvements. Different strategies are employed in the metabolic engineering of Clostridia that aim to enhance the solvent production, improve selectivity for butanol production, and increase the tolerance of Clostridia to solvents. The introduction and expression of a non-clostridial butanol producing pathway in E. coli is a most promising strategy for butanol biosynthesis. Several rigorous kinetic and physiological models for fermentation have been formulated, which form a useful tool for optimization of the process. Due to the lower butanol titers in the fermentation broth, simultaneous fermentation and product removal techniques have been developed to improve production economics. With the use of new strains, inexpensive substrates, and superior reactor designs, economic ABE fermentation may further attract the attention of researchers all over the world. The present review is attempting to provide an overall outlook on discoveries and strategies that are being developed for commercial n-butanol production.
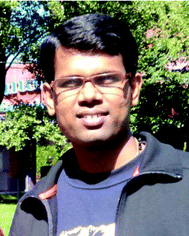 Sandip B. Bankar | Dr Sandip Balasaheb Bankar earned a Bachelor of Pharmaceutical Science from University of Pune, and Master of Bioprocess Technology from Institute of Chemical Technology (ICT), Mumbai India. He received a PhD in Bioprocess Technology in 2012. Soon after that he joined the Bioprocess Engineering Laboratory at Aalto University, School of Chemical Technology, Finland and is currently working as a post-doctoral research fellow. He has worked on range of biomolecules ranging from enzymes, polymers, carotenoids, therapeutic proteins to biofuels and biotransformation. His research interest broadly concern the Bioprocess Engineering of value added metabolites and lignocellulosic biofuels. |
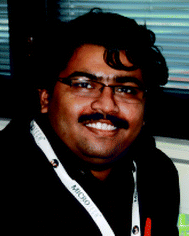 Shrikant A. Survase | Dr Shrikant Anurath Survase is a PhD graduate from Institute of Chemical Technology (formerly UDCT), Mumbai, India. He did his Bachelor in Pharmacy (B. Pharmacy) from University of Pune and M.Tech. (Bioprocess Technology) from ICT. He joined as a postdoctoral researcher at Department of Biotechnology and Chemical Technology, Aalto University in April 2010. He has worked with various biomolecules, enzymes, biofuels and organic acids. He has published over 33 research articles, 8 reviews and 2 book chapters. He also has 14 international conference presentations. His research areas include bioprocess development, biofuels, downstream processing and metabolic engineering. |
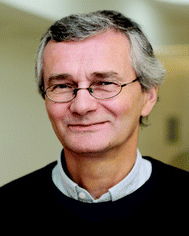 Heikki Ojamo | Dr Heikki Ojamo (born in 1953) is Professor of Bioprocess Engineering at Aalto University, School of Chemical Technology, Department of Biotechnology and Chemical Technology in Espoo Finland. Prof. Ojamo got his M.Sc. (Tech.) and D.Sc. (Tech.) degrees in Helsinki University of Technology Finland, which was one of the predecessors of Aalto University. He has worked for 35 years within Biotechnology, Research & Development and Education, first at the Technical Research Centre of Finland (VTT) and then at Cultor Oy (later Danisco), Medicel Oy, Espoo-Vantaa University of Applied Science and as a professor at the University of Oulu. |
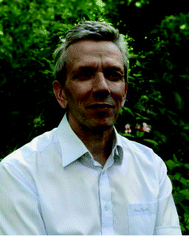 Tom Granström | Dr Tom Granström is a Senior Research Fellow and a group leader at Bioprocess Engineering Laboratory, Aalto University, Finland. His current industry and academia research projects include metabolically engineered Clostridia acetobutylicum and Lactobacillus to produce isopropanol and butanol respectively and cellulosic ethanol production. He has worked and studied in the UK, The Netherlands, USA and Japan. He is the principal lecturer of ‘Lignocellulose Biotechnology’ course at Aalto University and he has over 40 peer reviewed articles, 60 posters/public presentations, 3 book chapters and 3 patents. Currently, he is acting as a senior expert in cellulosic ethanol development processes in the industry. |
1. Introduction
The rapid depletion and environmental aspects of fossil fuels and highly fluctuating market prices have made the quest for alternative fuels a high priority. Over the past more than two decades, several alternative liquid fuels have been investigated, which can either completely replace the petroleum derived fuels (gasoline and diesel) or be blended with petroleum fuels in some proportion, without modifying the vehicle engines.1–3 First generation biodiesel although a popular biofuel, fails to replace petro-diesel completely, unless significant changes to the engine are configured.4,5 The low energy content (or heat of combustion) of ethanol influences the economy of the blended fuel. Moreover, given its solubility characteristics, ethanol is likely to separate from gasoline in the presence of water, which introduces some operational problems. Butanol isomers (n- or iso-butanol), overcome most of the limitations which are mentioned here. Besides being a potential biofuel, butanol is also a valuable C4 feedstock for chemical synthesis (esters, ethers, acetates, and plasticizers), as well as a solvent. The current international price of bulk grade butanol is approximately $4 per gallon (liquid fuel) with a worldwide market of 350 million gallons per year.6 The conventional chemical processes for butanol synthesis include the oxo process, wherein synthesis gas is reacted with propylene and hydrogenated subsequently to produce butanol. Table 1 lists and compares some common properties of various alternative liquid fuels. It can be seen that the properties of butanol match more closely with gasoline than the other listed fuels.
Table 1 Comparison of properties of different alternate liquid fuels6
Fuel |
Gasoline |
n-Butanol |
Isobutanol |
Ethanol |
Methanol |
Diesel |
Biodiesel |
Energy density (MJ l−1) |
32 |
29 |
29 |
19.6 |
16 |
39 |
31–33 |
Vapor pressure (kPa) at 20 °C |
0.7–207 |
0.53 |
1.17 |
7.58 |
12.8 |
Less than 0.07 |
Less than 0.07 |
Vapor pressure of mixture with gasoline (kPa) |
53.8–103.4 |
44.1 |
46.9 |
138 |
800 |
— |
|
Air : fuel ratio |
14.6 |
11.2 |
— |
9.0 |
6.5 |
— |
|
Heat of vaporization (MJ kg−1) |
0.36 |
0.43 |
— |
0.92 |
1.16 |
— |
— |
Research octane number |
91–99 |
96 |
— |
129 |
129–134 |
— |
— |
Motor octane number |
81–89 |
78 |
112 |
102 |
97–104 |
— |
— |
Cetane number |
— |
— |
— |
54 |
|
45 |
4958 |
Freezing temperature (°C) |
Less than −60 |
−89.5 |
−108 |
−114.5 |
−97.6 |
−30 to −9.9 |
7.5 to −16 |
Hygroscopicity |
Low |
Low |
Low |
High |
High |
Very low |
Very low |
Compatibility with existing infrastructure |
Yes |
Yes |
Yes |
No |
No |
Yes |
No |
Apart from the merits listed in Table 1, butanol also offers some advantages over ethanol and methanol i.e. (a) it can be blended to a varied ratio with gasoline as well as diesel directly in the refinery without any additional infrastructure. (b) Easy transportation and less corrosion through pipelines because of low vapor pressure. (c) The air
:
fuel ratio for butanol is close to that of gasoline which is permissible in existing vehicle engines. The complete replacement of gasoline by butanol would require an enhancement of the air
:
fuel ratio, but blends up to 20% of butanol can be directly used in engines without any modifications.7 (d) The heat of vaporization of butanol is slightly higher than that of gasoline. Hence, the butanol-blended engine does not exhibit the cold start problem as seen in methanol or ethanol blended gasolines.7 (e) The low solubility of butanol in water reduces the potential for groundwater contamination.
Different routes of biosynthesis of butanol from various biological substrates such as sugars, starch, and biomass are available.6 Biologically produced n-butanol is popularly known as ‘biobutanol’. The most prevalent and historical route to biobutanol production is by acetone–butanol–ethanol (ABE) fermentation with the solvent-producing strains of Clostridium sp.8,9 The conventional substrates for the ABE fermentation have been corn starch or molasses; however, as reviewed subsequently in this paper, several alternative substrates have also been considered.
1.1. Chemical synthesis of butanol
Oxo synthesis, Reppe synthesis, and crotonaldehyde hydrogenation are considered as the three most important processes for the chemical butanol industry.8,9 Oxo synthesis (hydroformylation), involves the addition of carbon monoxide and hydrogen to a carbon–carbon double bond using catalysts such as Co, Rh, or Ru substituted hydrocarbonyls.10 In the Reppe process, propylene, carbon monoxide and water are reacted in the presence of a catalyst to produce butanol at low temperature and pressure.11 Crotonaldehyde hydrogenation consists of aldol condensation, dehydration, and hydrogenation.11
2. Biobutanol
Fermentative ABE production prospered during the early 20th century after Pasteur discovered it from anaerobic cultivation in 1861. In 1945, two thirds of industrially used butanol was produced by fermentation in USA and the ABE production evolved as the second largest industrial fermentation process in the world after ethanol. However, due to progression of the petrochemical industry, low solvent yields and the increase in feedstock cost, the ABE fermentation process had lost its competitiveness by the 1960s.12 Research and development of the ABE process continued and during the 1980s and 1990s studies at pilot plants were carried out in several countries. Few countries such as China, South Africa and Russia developed their ABE process industry with Clostridium strains from starch based feedstocks.13 In the past few years China has restarted ABE production including at least 11 production plants which are in the operation and others are under construction or at the start up stage.14,15
2.1. Applications of butanol
An estimated of 10–12 billion pounds of butanol is produced annually, which accounts for a 7–8 billion $ market at the current price.16 Butanol has a projected market growth of 3% per year.17 The most important application of butanol receiving renewed attention is its use as a fuel additive or as a direct replacement of gasoline. In the automotive fuel market, it offers substantial advantages over petroleum gasoline. Butanol has been shown to be compatible with OEM (original equipment manufacturer) gasoline engines without modification and exhibits comparable performance.18 It is also compatible with the existing fuel pipeline infrastructure. Under current US Environmental Protection Agency (EPA) regulations, butanol can be added as an oxygenate to gasoline in concentrations up to 11.5% (v/v). Butanol is safer to handle than gasoline, less flammable (lower vapor pressure and higher flash point) and is miscible with gasoline in any ratio. It is non-hygroscopic, non-corrosive, and proves an overall low order of toxicity.19 Butanol has a volumetric energy density of 29.2 MJ l−1, which is comparable to that of gasoline (32.5 MJ l−1), and much higher than that of ethanol (19.2 MJ l−1) (Table 1). Motor gasoline consumption in the USA is about 370 million gallons per day representing a huge fuel market.20 The Energy Independence and Security Act of 2007 mandates that the volume of renewable fuels sold in the USA must be 30 billion gallons by 2020, of which 10.5 billion gallons must be of lignocellulosic origin.21
The other direct application of butanol is as an industrial solvent or co-solvent, mainly for surface coatings (resins, lacquers, varnishes, waxes). About 50% of butanol production is used in the form of butyl acrylate and methacrylate esters used in latex surface coating, enamels and lacquers.17 Other important derivatives of butanol are butyl glycol ether, butyl acetate and plasticizers. Butanol is also an excellent diluent for brake fluid formulations and solvent for the manufacture of antibiotics, vitamins and hormones.
2.2. Microorganisms
Butanol (along with, acetone, ethanol, and isopropanol) is naturally formed by a number of Clostridia.22Clostridia are typically strict anaerobes and rod-shaped, spore-forming Gram positive bacteria. Solventogenic Clostridia can utilize a large variety of substrates from monosaccharides including many pentoses and hexoses to polysaccharides.23 Among many solventogenic Clostridia, C. acetobutylicum, C. beijerinckii, C. saccharobutylicum, and C. saccharoperbutylacetonicum are primary solvent producers.24C. beijerinckii NCIMB 8052, C. saccharobutylicum, and C. saccharoperbutylacetonicum were originally designated as C. acetobutylicum.24
The Clostridial solvent production is biphasic fermentation, which is detailed in subsequent sections of this paper (Fig. 1). The first phase is the acidogenic phase, during which the acid forming pathways are activated, to produce acetate, butyrate, hydrogen, and carbon dioxide as major products. The acidogenic phase usually occurs during the exponential growth phase of the Clostridia.25,26 The latter phase is the solventogenic phase wherein acids are reassimilated and used in the production of acetone, butanol and ethanol (or isopropanol in some C. beijerinckii strains). The transition from the acidogenic to solventogenic phase is the result of an intense change in gene expression pattern.27 The biosynthetic pathways involving acidogenic and solventogenic phase for n-biobutanol production are discussed scrupulously in subsequent sections of this paper.
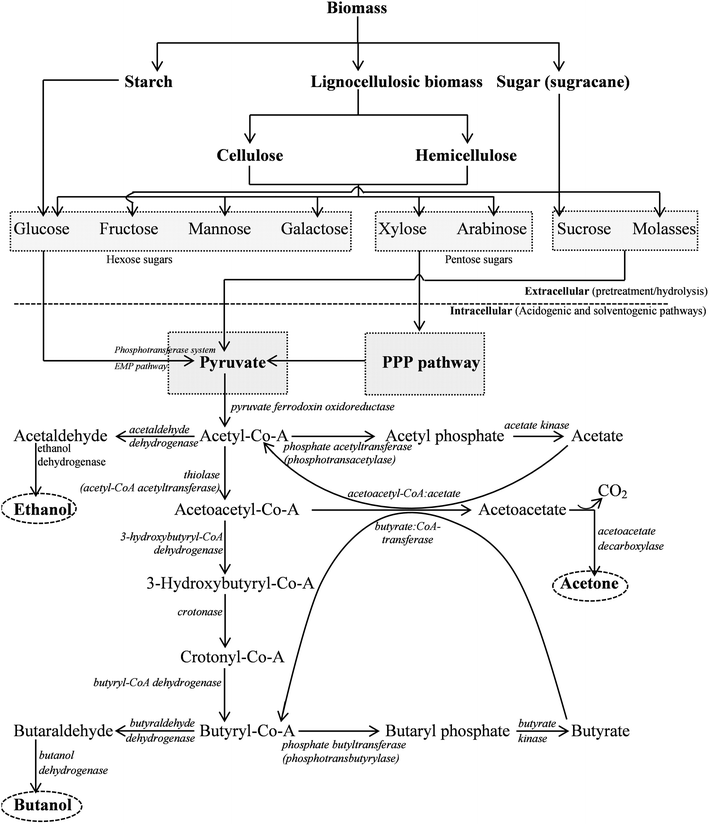 |
| Fig. 1 The metabolic pathway of acetone–butanol–ethanol (ABE) fermentation by C. acetobutylicum.23,41,116 | |
2.3. Biomass for biobutanol
Low conversion rates of conventional fermentative technologies which in turn result in the economically unviable large-scale production is the key reason for less attracted market interest of biobutanol. However, a number of biotechnology ventures with proprietary approaches to microbial strain selection and engineering, feedstock handling, bioreactor management, and product separation have emerged as a new generation of butanol producers (Butamax, Gevo, Green Biologics, etc.). These are established as a major force in the renewables market. Biomass is the fourth largest source of the energy in the world after coal, petroleum and natural gas, and it is also a carbon neutral resource over its life cycle. Although, some plants that are rich sources of starch such as corn, soybeans, and potatoes could be used to make liquid biofuels, this has been criticized for diverting food away from the human food chain, leading to a food versus feed/energy war. The fermentation feed substrate is also an important factor influencing the cost effectiveness of biobutanol production.
A major advantage of using biomass substrates for fuel production is their renewability. Biomass is a complex mixture of organic materials, but the main components of plant biomass are polymeric carbohydrates (approximately 75% of dry weight) including celluloses and hemicelluloses with lignins which function in imparting strength to the plant structure and holding the composition together.28Clostridia can secrete numerous enzymes that facilitate the breakdown of polymeric carbohydrates into sugar monomers for biobutanol production.29 However, the acidic or enzymatic hydrolysis of lignocellulosic materials is essential to convert them into monosaccharides before using them as substrates in ABE fermentation. Clostridia are not cellulolytic bacteria and hence cannot utilize cellulose as a carbon source. However, cellulosomes are multienzyme complexes, which have high activity against crystalline cellulose, are being analyzed in Clostridia.30 The lignocellulosic biomass is the most abundant renewable resource on the earth for biofuel production.31,32 Countries like India alone generate over 370 million tonnes of biomass every year directly from plants, rice husk from rice mills, saw dust from saw mills, bagasse from sugar mills etc.33 Experimentally, the potential of numerous lignocellulosic feedstocks such as wood forestry residues, corn stover, wheat straw, corn fibers, barley straw, and switchgrass have been tested for ABE fermentation.34–42
2.4. Biochemistry of the ABE fermentation
In the natural state, bacterial butanol production is carried out exclusively by the members of the genus Clostridia including C. acetobutylicum, C. beijerinckii, C. saccharoperbutylacetonicum, C. saccharoacetobutylicum, C. aurantibutyricum, C. pasteurianum, C. sporogenes, C. cadaveris, C. tetanomorphum.43 The most commonly considered substrates for the Clostridial cultures include fibrous biomass containing hemicellulose and cellulose (e.g., wheat straw, rice straw); starchy biomass (such as ground corn and whey permeate); and fruits and vegetables containing fructose, glucose and xylose as the basic components. Laboratory-based media for the culture growth have normally been semi-defined and defined wherein the main carbohydrate source is supported by various vitamins and minerals depending on the microbial cultures. The optimum temperature for the ABE fermentation is between 30 and 40 °C, while the initial pH of the fermentation broth, is 6.8–7, and it drops down to 4.5–5 during the acidogenic phase.
3. Fermentative production of biobutanol
A typical ABE fermentation using C. acetobutylicum yields acetone, butanol and ethanol in the ratio of 3
:
6
:
1. A high concentration of acetyl-CoA is needed to increase the overall solvent production and hence the quantity of acetyl-CoA plays an important role in determining the ratio of C3 + C4 products to C2 products.44,45 ABE fermentation undergoes an acidogenic phase in the exponential growth phase, followed by a switch to the solventogenic phase at the end of the exponential growth phase. The acidogenic phase is accompanied by a higher butyrate concentration than acetate in the fermentation broth. The formation of butyrate resolves an issue of redox equilibrium – the NADH produced during glycolysis is consumed only in the butyrate, and not in the acetate formation pathway. This results in a higher concentration of butanol accumulation than ethanol in the fermentation, since most of the butyrate and acetate is converted to butanol and ethanol in the solventogenic phase, respectively. Development of a fermentation system to produce a higher butanol yield and productivity in a cost effective way has been practiced by various researchers. Recent developments in the use of various types of biomasses for ABE production are discussed in subsequent sections.
3.1. Batch fermentation
3.1.1. Fermentation with biomass hydrolysate.
Even though batch fermentation processes have several disadvantages such as time consumption during sterilization of bioreactors, major down time, long lag phase, solvent inhibition and low productivity; they are traditional and are still useful in many respects. The use of various cheap feedstocks such as Jerusalem artichokes, cheese whey, apple pomace, algal biomass, sulfite waste liquors, and various hydrolysates for the production of biobutanol has been reviewed and described by Jones and Woods.23
Continuing our previous work to update on recent developments, the use of various sustainable feedstocks by various researchers for ABE fermentation is reviewed in Table 2.46 Jesse et al.47 performed the fermentation of waste streams such as starch-based packing for peanuts and agricultural waste as a source of fermentable carbohydrates using C. beijerinckii BA101 and produced 21.7 g l−1 and 14.8 g l−1 ABE, respectively. The use of enzyme hydrolysate of palm oil mill effluent has been reported as a growth medium substitute, a fermentation substrate, and as a source of nitrogen and micronutrients for the production of ABE by Hipolito et al.48 Agricultural wastes such as wheat bran, rice bran, maize stalk, barley straw etc. were studied by various researchers as sugar sources. Different pretreatments and hydrolysis methods were also studied for the respective feedstocks. Qureshi et al.35–37 studied five different processes to produce ABE from wheat straw (WS) by Clostridium beijerinckii P260. They found simultaneous saccharification and fermentation with agitation by gas stripping to be efficient but suggested that fed batch operation with glucose supplementation can make further improvements in solvent production and yield. Lee et al.49 used rice bran and defatted rice bran to get the highest butanol production (12.24 g l−1) from the hydrolysate after acid and enzyme treatment with supplementation in the production medium. Wheat bran, a by-product from the wheat processing industry was shown to be a potential substrate for ABE production. Liu et al.50 used acid hydrolysed wheat bran and produced 53.1 g l−1 monomeric sugars from 100 g l−1 of wheat bran. Qureshi et al.38 studied barley straw hydrolysate (BSH) in four different ways such as (i) undiluted/untreated BSH, (ii) diluted BSH (20 mL BSH and 20 ml sterile distilled water), (iii) diluted BSH with equal amount of wheat straw hydrolysate (WSH: 20 ml BSH and 20 mL WSH), and (iv) overlimed BSH. The total solvents produced in the various fermentation systems were 7.09, 17.42, 17.18, and 26.64 g l−1, respectively suggesting significance of overliming in reducing the effect of inhibitors. Qureshi et al.39 further performed similar experiments with corn stover and switchgrass hydrolysates and concluded the importance of dilution and overliming to get comparable ABE solvent production.
Table 2 Different feedstocks and hydrolysis methods used along with maximum solvents and productivities achieved46
Hydrolysis method |
Substrate |
Yield (g g−1)/productivity (g l−1 h−1) |
Total ABE (g l−1) |
Ref. |
Butanol yield and productivity.
|
None |
Waste packing for peanuts |
0.37/0.20 |
21.7 |
47
|
Date fruit |
0.41/0.09 |
6.2 |
243
|
|
Dilute sulfuric acid + enzyme |
|
Wheat straw |
0.60/0.42 |
25 |
244
|
Wheat straw |
0.41/0.31 |
21.42 |
36,37
|
Barley straw |
0.43/0.39 |
26.64 |
38
|
Corn stover |
0.44/0.31 |
26.27 |
39
|
Switchgrass |
0.39/0.17 |
14.61 |
39
|
Rice straw |
1.04a/0.017a |
3.0a |
109
|
|
Dilute sulfuric acid |
|
Corn fiber |
0.39/0.10 |
9.3 |
34
|
Wheat bran |
0.32/0.16 |
11.8 |
50
|
Corn fiber |
0.36/0.14 |
10.1 |
61
|
Corn fiber |
0.32/0.14 |
10.6 |
62
|
|
Enzyme |
|
Palm oil mill effluent + sago starch |
0.40/0.10 |
14.38 |
48
|
Cassava bagasse |
0.39/0.62 |
33.87 |
72
|
Corn cob residue |
0.32/0.33 |
16 |
245
|
Sago pith residue |
0.30/0.12 |
8.84 |
246
|
Ammonium fiber expansion + enzyme |
Dried distillers' grains and soluble (DDGS) |
0.34/0.14 |
10.4 |
247
|
Dilute HCl + enzyme |
Rice bran and defatted rice bran |
0.31/0.26 |
12.24 |
49
|
HCl |
Jatropha seed cake |
0.45/0.26 |
18.4 |
54
|
Hot water extraction + sulfuric acid |
Sugar maple wood |
0.22/0.15 |
11.0 |
53
|
SO2–ethanol–water |
SO2–ethanol–water (SEW) spent wood liquor |
0.20/0.09 |
8.79 |
51
|
The SO2–ethanol–water (SEW) spent liquor obtained by fractionation of spruce chips was fermented in batch culture by dilution (4 times) and supplementation with added glucose (35 g l−1) resulted in 8.79 g l−1 ABE solvents.51 Wang and Blaschek,52 used response surface methodology to optimize pH, sugar concentration and agitation in batch fermentation of maize stalk juice. The optimum conditions of pH 6.7, a sugar concentration 42.2 g l−1 and an agitation rate 48 rpm were estimated to give a maximum butanol yield of 0.27 g g−1 sugar. In yet another study, sugar maple wood was subjected to hot water extraction followed by membrane filtration and hydrolyzed with sulphuric acid. The hydrolysate was further treated with nanofiltration and overliming to remove inhibitors. Overliming treatment significantly improved the butanol concentration from 0.8 to 7 g l−1.53 Isar et al.54 showed the potential of Jatropha seed cake (JSC) as a substrate for biobutanol production. They reported a butanol titer of 13.2 g l−1 in 120 h using acid-treated JSC (7% w/v) with supplementation of suitable medium components. They further successfully scaled up the process to 15 liter and improved butanol titer to 18.6 g l−1 after 72 h of fermentation.
3.1.2. Hydrolysates detoxification.
Various compounds such as salts, furfural, hydroxymethyl furfural (HMF), acetic, ferulic, glucuronic, p-coumaric acids, and some other phenolic compounds are reported to be formed during a pretreatment and hydrolysis of agricultural biomass. Ezeji et al.41 reported that, p-coumaric and ferulic acids at 0.3 g l−1 affected growth and ABE production by C. beijerinckii BA101 whereas, furfural and HMF stimulated the growth of the microorganism and ABE production. Qureshi et al.55 reported similar findings concluding that furfural and HMF formed in the wheat straw hydrolysate (WSH) are enhancing the ABE productivity, specific productivity, and ABE concentration. Among various acid hydrolysis products screened, the inhibition rate of butanol production was 88.1%, 82.4%, 22.1%, 9.8% and 4.6% when 0.5 g l−1 formic acid, p-coumaric acid, ferulic acid or 1 g l−1 furfural and HMF respectively were present in the medium.56 Cho et al.57 described the peroxidase-catalyzed detoxification method for p-coumaric acid, ferulic acid, 4-hydroxybenzoic acid, vanillic acid, syringaldehyde, and vanillin, and evaluated the inhibitory effects of the detoxified solution on butanol production by Clostridium beijerinckii. Qureshi et al.38,40 and Survase et al.51 reported overliming and dilution as one of the ways to make hydrolysates fermentable. Mu et al.58 reported the separate fermentation of glucose and xylose obtained by the steam explosion method and enzymatic hydrolysis of corn stalk as a novel technology for improved ABE production. They also found Ca(OH)2 treatment to be the best detoxification method as compared to NaOH and Na2SO3. The fermentability of enzymatically hydrolyzed steam-exploded corn stover (SECSH) was improved using activated charcoal treatment and alkaline peroxide treatment, both mainly affecting the phenolic compounds. The ABE fermentation was found to be inhibited above 1.77 g l−1 soluble lignin in the SECSH-based medium whereas fermentation was improved when concentration was less than 0.89 g l−1.59 Cai et al.60 reported the use of pervaporation with a PDMS membrane for the detoxification of sweet sorghum bagasse (SSB) hydrolysate before the fermentation to remove 94.5% furfural. Guo et al.61,62 have developed C. beijerinckii which was tolerant to inhibitors (total phenolic compounds) when corn fiber hydrolysate was used as a substrate.
3.1.3. Immobilization materials in fermentation.
The presence of adsorption material and immobilization of the solvent producing strain have been reported to be beneficial by many researchers in the literature. The immobilization strategy is claimed to improve the stability and solvent tolerance of the microorganisms. There are various reports on different types of immobilization materials ranging from synthetic to biodegradable polymers and fibers. Tripathi et al.63 suggested agarose–alginate cryogel beads after comparison with other potential support matrices such as coconut fibers, brick pieces and burnt coal. Efremenko et al.64 showed that the immobilization of Clostridium cells using poly(vinyl alcohol) cryogel changed the ratio of ABE solvents (4
:
12
:
1) produced resulting in higher butanol titers. Various immobilization matrices were screened in batch fermentation.63,65,66 Survase et al.66 recently reported that the addition of a support matrix improves the substrate consumption and its conversion to solvents. Coconut fibers and wood pulp were found to be the most promising support matrices. Shamsudin et al.65 investigated the ABE production by Clostridium saccharoperbutylacetonicum N1-4 using different immobilization materials and concluded that passive immobilization on polyurethane foam increased the production by 215.12%. Napoli et al.67 screened various carriers including silica gel, Tygon, Teflon, PVC and glass beads for better biofilm formation by observing SEM after 1 week.
3.2. Fed-batch fermentation
As compared to batch culture, fed-batch fermentation has some advantages such as the use of highly concentrated substrate, which can reduce hydraulic load and wastewater generated in the process. Li et al.68 compared different modes of fermentation such as batch, fed-batch and continuous with pH-control at 4.5 and claimed that the time needed for passing from acidogenesis to solventogenesis was an intrinsic hindrance to higher butanol productivity. However, the fed-batch mode was not suggested for ABE solvent production. The continuous lactic acid and glucose feeding produced 15.5 g l−1 butanol at a rate of 1.76 g l−1 h−1 using a fed-batch culture with a pH maintained at 5.5.69 They suggested that different acids such as lactic acid, acetic acid and butyric acid can be used as the substrates for butanol production. Similar findings were reported by Tashiro et al.70 wherein they demonstrated enhanced productivity of butanol by feeding butyric acid along with glucose in pH stat fed-batch culture. The maximum butanol concentration of 16 g l−1 was achieved with a 72% higher productivity than in a conventional batch process. Qureshi et al.35 demonstrated that simultaneous hydrolysis of wheat straw and fermentation to butanol is possible if the straw is fed with some extra sugar. They could achieve 100% hydrolysis of WS to monomeric sugars and this also helped in improving the productivity by 16% during a fed-batch experiment of 533 h. When Guo et al.62 used non-detoxified sulphuric acid hydrolysate of corn fiber as the substrate in a fed-batch fermentation, a total ABE of 12.9 g l−1 was produced by C. beijerinckii RT66, with yield of 0.35 g g−1 sugar and productivity of 0.18 g l−1 h−1 in 72 h.
Ezeji et al.71 claimed that in the integrated fed-batch fermentation and product recovery system, solvent productivities were improved to 400% of the control batch fermentation. A total of 500 g of glucose was utilized in 201 h to produce 232.8 g solvents with an average solvent yield and productivity of 0.47 g g−1 and 1.16 g l−1 h−1, respectively. The highly concentrated cassava bagasse hydrolysate containing mainly glucose using the hyper-butanol-producing C. acetobutylicum JB200 produced 108.5 g l−1 ABE in a fed-batch fermentation with simultaneous butanol recovery by gas stripping in 263 h. The integrated fermentation process is claimed to be attractive because of a stable productivity and high butanol yield for an extended period with periodical nutrients supplementation.72
3.3. Continuous fermentation
Continuous fermentation has several advantages over batch and fed-batch fermentations such as minimizing downtime and the lag phase. Additionally, the time needed for sterilization and inoculation substantially lowers the productivity. The modes of continuous fermentation include suspended cell, cell recycling and immobilized cell fermentation. Reactor configurations for continuous production of chemicals can be a continuously stirred tank reactor (CSTR) with or without immobilization material, packed bed reactor (PBR), fluidized bed reactor (FBR), airlift reactor, upflow anaerobic sludge blanket reactor, or any other suitable configuration.73
3.3.1. Single stage reactors.
In a chemostat CSTR, feeding of the production medium and product removal are at the same rate to keep broth volume constant. CSTR mainly include suspended cell fermentations which have been reported for continuous ABE production.8,29,68,74 The use of saccharified degermed corn in suspended cell continuous fermentation achieved a total ABE concentration up to 14.16 g l−1 during fermentation run for 504 h.29 The suspended cell continuous fermentation could not reach high productivities because of non-applicability at higher dilution rates. The operation of such reactors at a higher dilution rate caused cell wash out.8,68,74 To overcome this limitation, CSTRs with an adsorbent material such as porous polyvinyl alcohol8 and wood pulp74 to attach the cells on and retain the in the reactor are reported in the literature. When cells are retained the limitation D < μmax can be circumvented. During continuous butanol production using porous polyvinyl alcohol immobilized cells; the overall butanol productivity and yield were 0.40 g l−1 h−1 and 0.44 g g−1 of glucose, respectively, which were approximately twice the values in a suspended free-cell continuous reactor.8 When wood pulp was added to the bioreactor as a cell holding material to prevent the cell wash out; higher dilution rates could be employed. This increased the solvent (isopropanol and butanol) productivity from 0.47 to 5.52 g l−1 h−1 with a yield of 54% from glucose.74 The average butanol concentrations with brick immobilized cells and suspended cells were 8.07 and 4.56 g l−1, respectively. However, the ratio of butanol to the total solvents (ABE) was similar suggesting that the cell immobilization did not alter the metabolic pathway.75
3.3.2. Single stage reactors with cell recycling.
To overcome the low cell density (approximately 2–3 g l−1) in traditional ABE fermentation, continuous fermentation processes with cell recycling and retention have been successfully applied.76,77 This approach helped to maintain high productivities for a long period without cell degeneration.76 A continuous fermentation of C. pasteurianum MBEL-GLY2 (hyper-producing strain) with cell recycling was carried out to get the ABE and the butanol productivity of 8.3 and 7.8 g l−1 h−1 respectively, at a dilution rate of 0.9 h−1.77 Zheng et al.78 have reported a continuous butanol production system from glucose and xylose with high cell-density generated by cell recycling. Cell recycling increased cell concentration to 17.4 g l−1 which increased butanol productivity to 1.20 g l−1 h−1 from 0.529 g l−1 h−1 at a dilution rate of 0.26 h−1.
3.3.3. Multistage reactors.
Multistage reactors were reported in the literature to facilitate increased substrate consumption. There are some reports on integrated solvent removal modules to further prevent inhibition by produced solvents and improve volumetric productivity and yields. Bankar et al.79,80 optimized continuous ABE fermentation using a two stage chemostat system and two stage column reactors integrated with a liquid–liquid extraction module and reported to improve the substrate consumption and total ABE production. An overall solvent production of 25.32 g l−1 was observed with volumetric solvent productivity and solvent yield of 2.5 g l−1 h−1 and 0.35 g g−1, respectively.79 The maximum solvent productivity (10.85 g l−1 h−1) and the solvent yield (0.38 g g−1) were obtained at a dilution rate of 1.0 h−1 in two stage immobilized column reactors integrated with liquid–liquid extraction module.80 Setlhaku et al.81 studied an innovative two stage process for ABE fermentation where the first stage was operated in a continuous mode and the second stage as a fed-batch. Intermittent gas stripping was coupled with the second stage to produce 59 g l−1 of butanol and 73 g l−1 of total ABE solvents in the condensate. With ex situ pervaporation using a PDMS membrane at a temperature of 37 °C and pressure of 10 mbar, 167 g l−1 butanol and 269 g l−1 ABE was analysed in the permeate. Corn stover hydrolysate (CSH) and cane molasses as substrates were studied by using four stage continuous reactors. Using cane molasses as substrate, 13.75 g l−1 total ABE solvents and productivity of 0.439 g l−1 h−1 were achieved at a gradient dilution mode of 0.15–0.1 h−1. In a continuous fermentation using CSH as substrate, total solvent titer of 11.43 g l−1 and productivity of 0.429 g l−1 h−1 were reached at a dilution rate of 0.15 h−1.82
3.3.4. Packed bed reactors.
Packed bed reactors (PBRs) are packed with suitable immobilization material to form a biofilm. They are fed with a nutrient-rich production medium usually from the bottom. PBRs suffer from excessive cell growth followed by blockage but the reaction rates are much higher as compared to batch reactors.73 In the past, various materials such as bonechar,83 coke,84 beechwood shavings,85 chitosan, carrageenan and sodium alginate matrices have been used as the support materials.86 Recently, more immobilization materials were studied for PBR continuous reactors as listed in Table 3. Qureshi et al.87 studied a packed bed reactor using clay brick as an immobilization material and reported the highest productivity of 15.8 g l−1 h−1 at a dilution rate of 2 h−1. However, the substrate utilization was only 33% at this dilution rate. Qureshi et al.88 scaled up the biofilm reactor with high volumetric productivities, the highest being 16.13 g l−1 h−1 at a dilution rate of 2 h−1 with reactor operation time of 2302 h. To increase sugar utilization, the reactor effluent was suggested to be recycled by Lienhardt et al.89 This approach resulted in complete sugar utilization with higher productivity up to 10.2 g l−1 h−1 at a dilution rate of 1.5 h−1. The highest reactor productivity (16.2 g l−1 h−1) was reported at a dilution rate of 2.0 h−1.
Table 3 Summary of recent continuous fermentation reports for ABE production46
|
Yield (g g−1)/productivity (g l−1 h−1) |
Total ABE (g l−1) |
Ref. |
Butanol.
Polyvinyl alcohol.
|
Single stage reactor with free cell cultivation |
|
0.11/0.37 |
6.85 |
75
|
0.23/0.89 |
8.85 |
68
|
0.28/0.47 |
5.26 |
74
|
—/0.30 |
10.12 |
29
|
0.24/0.22a |
7.10a |
8
|
—/1.85 |
9.27 |
76
|
|
Single stage reactor with cell recycle and bleeding |
|
—/8.3 |
9.2 |
77
|
—/7.55 |
12.9 |
76
|
—/3.32a |
4.26a |
78
|
|
Immobilized cell reactor |
Brick |
0.19/1.21 |
11.28 |
75
|
Wood pulp |
0.29/5.52 |
9.20 |
74
|
PVAb |
0.44/0.40a |
13.4a |
8
|
Wood pulp |
0.27/4.86 |
7.59 |
51
|
Wood pulp |
0.28/12.14 |
8.09 |
66
|
Fibrous matrix |
0.42/4.6a |
5.11a |
248
|
Corn stalk |
0.32/5.06 |
5.10 |
249
|
Brick |
0.30/2.01 |
6.29 |
88
|
Ceramic D-21beads |
—/1.0 |
7.73 |
250
|
Tygon™ rings |
0.28/5.0 |
5.19 |
67
|
Tygon™ rings |
0.26/2.66a |
4.93a |
91
|
Brick |
0.38/15.8 |
7.9 |
87
|
Brick |
—/16.1 |
8.10 |
89
|
|
Two stage immobilized cell reactor |
None |
0.32/0.84 |
7.63 |
74
|
Sugarcane baggase |
0.35/2.47 |
12.37 |
79
|
Wood pulp |
0.38/10.85 |
13.60 |
80
|
Survase et al.51,66,90 used SEW spent liquor from spruce chips and a sugar mixture identical to wood hydrolysate as a feed on wood pulp immobilized PBR to report the highest productivity of 4.86 g l−1 h−1. PBR reactor with Tygon rings as an immobilization material and lactose as a feed was reported by Napoli et al.67 to get maximum productivity of 5 g l−1 h−1. Tygon rings have recently also been used as carriers with un-supplemented cheese whey as renewable feedstock. Under optimized conditions, volumetric butanol productivity, concentration and yield were 2.66 g l−1 h−1, 4.93 g l−1 and yield 0.26 g g−1, respectively.91
4. Butanol inhibition
As can be seen from the previous sections, during repeated batch, sub-culturing or continuous culturing of Clostridia, butanol formation is known to be inhibitory to the producing microorganisms. This is the most crucial hurdle for butanol fermentation to commercialize the process, as bacterial strains rarely tolerate more than 2% butanol.92 Solvent toxicity limits the solvent yield, solvent productivity and leads to a low concentration of solvents in the fermentation broth. A higher butanol concentration induces adverse changes in phospholipid and fatty acid composition in the cell membrane of C. acetobutylicum. This adverse change destroys the unsaturated and saturated fatty acids ratio (U/S) and also decreases the specific interaction of alcohol with individual components of lipids.93 Specifically, butanol (harmful organic solvent) enters into the cytoplasmic membrane and alters the membrane structure. It also disrupts the number of physicochemical characteristics such as preferential solute transport, membrane permeability, maintenance of the proton motive force (or maintenance of intracellular pH), intracellular ATP level, glucose uptake, and conformation and activity of intrinsic membrane proteins. An increment of 20–30% of fluidity in membrane is observed with only 1% butanol exposure.94,95 The butanol-induced degeneration is more severe than acetone induced degeneration.96,97
Researchers all over the world have endeavored to reduce the toxicity of butanol during fermentation at the molecular level as well as by developing an advanced bioprocess. The butanol tolerance to the clostridial microorganisms was facilitated by various techniques as discussed in the subsequent sections of this review. Several butanol tolerant strains were developed from a classical butanol producer organism (C. acetobutylicum) using mutagenesis and by genetic manipulation.92 One attractive achievement in process development, namely extractive fermentation is also being studied to reduce the butanol-induced inhibition effect.79,80 In this approach, production as well as selective removal of butanol was carried out simultaneously to maintain a low concentration of butanol in the fermentation broth. The common online butanol removal techniques are adsorption, liquid–liquid extraction, perstraction, reverse osmosis, pervaporation, and gas stripping, which could be integrated with ABE fermentation45 and partly already dealt with in this text.
4.1. Strain degeneration in solventogenic Clostridia
Strain degeneration is a general challenge experienced in the fermentation industry which includes the loss of capacity to produce solvents when Clostridia are prone to sporulate. Solventogenic Clostridia lose their productivity if the cells are maintained in the vegetative state for a prolonged period of time.98 Strain degeneration is a result of genetic changes and is different from loss of ability to produce solvents under unfavorable cultivation conditions. Degeneration is also different from the loss of the solvent production ability due to a mega-plasmid loss which is a common phenomenon for C. acetobutylicum. Thus the maintenance of cells in the vegetative state via re-passaging or continuous cultivation leads to an accumulation of degenerated cells within the culture, while the density of the culture and the rate of metabolism decrease.99 Compared to the non-degenerated cells, degenerated C. beijerinckii and C. saccharobutylicum cells are longer and thinner, and they form larger colonies with irregular shapes.98,100 Kashket and Cao,101 isolated a degeneration-resistant mutant of C. acetobutylicum NCIMB 8052. The longevity of the mutant was three times higher than that of the wild type strain. The disruption of a 486 bp regulatory RNA that is able to form a stable hairpin structure was speculated to be associated with the reduced tendency to degenerate.102 The development of mutants with a reduced level of degeneration along with an identification of the Spo0A factor as a main regulator of the solvent production is under the progress. Bacteriophage infection is another major problem in ABE fermentation. Among the approaches used to eliminate the negative effect of bacteriophage on Clostridia fermentation, the most successful and widespread involves the isolation of phage-resistant mutants or variants.103
5. Metabolic pathways
The fermentation process inherently relies on the level of metabolic activities of the organism. Major end products from the metabolic pathway activities of clostridial species (C. acetobutylicum and C. beijerinckii) are butanol, acetone, ethanol, acetic acid, butyric acid, CO2, and H2.45 Metabolic activities of the organism broadly observed in acid producing and solvent producing phases are briefly discussed in subsequent sections. Recently, it has been reported that the Clostridia secrete extracellular enzymes like amylase, glucosidase, glucoamylase, pullulanase, and amylopullulanase to digest complex polysaccharides into simple monosaccharides like glucose, xylose and arabinose.41,42 However, cellulolytic Clostridia were known to be extremely sensitive to oxygen and have low aero-tolerance control. The peroxide repressor (PerR) gene, which is responsible for regulating detoxification genes, was identified as a factor for the low aero-tolerance of C. acetobutylicum.104–106 Anaerobes (obligate/facultative) uptake sugars via the phosphoenolpyruvate-dependent phosphotransferase system.107,108 Generally, hexose sugars are metabolized by the Embden–Meyerhof pathway (EMP), whereas pentose sugars go through the pentose phosphate pathway (PPP) to produce pyruvate (Fig. 1). Glycolysis utilizes glucose (1 mol) and produces pyruvate (2 mol), whereas PPP results in the production of CO2 (6 mol). The PPP has several roles including use of nicotinamide adenine dinucleotide (NADH) for anabolism as well as formation of C3 and C7 intermediates. During glycolysis, energy is stored as adenosine triphosphate (ATP), whereas during PPP, energy is stored as NADPH. The most common substrate for the ABE fermentation is starch, which is converted to glucose following acid or enzymatic hydrolysis. Glucose (6 C) is first phosphorylated to glucose-6-phosphate, which is subsequently converted to pyruvate (3 C) via EMP. Other fermentation substrates containing hemicellulose or cellulose (e.g. fibrous biomass) can be converted to xylose and glucose, respectively, after hydrolysis.109 Glucose follows the metabolic pathway as explained earlier, whereas xylose (existing naturally in the form of D-xylose, an aldose) undergoes the ‘isomerase pathway’ in which the enzyme xylose isomerase convert D-xylose to D-xylulose. Furthermore, D-xylulose undergoes phosphorylation to form D-xylulose-5-phosphate which then dissimilates by means of transketolase and transaldolase through non-oxidative pentose phosphate. This results in the production of glyceraldehydes 3-phosphate and fructose-6-phosphate, which finally enters the EMP pathway for further conversion.23,110,111 Fermentation of hexose sugar (1 mol) results in generation of 2 mol of ATP and 2 mol of reduced NADH.23 ATP produced during the consumption of glucose results in the exponential growth of cells.
The pyruvate formed during the glycolysis/EMP pathway is cleaved by pyruvate ferredoxin oxidoreductase in the presence of co-enzyme A (CoA) producing CO2, acetyl-CoA, and reduced ferredoxin. Conversion of acetyl-CoA to acetate is achieved by the enzymes phosphate acetyltransferase and acetate kinase, whereas conversion of butyryl-CoA (formed through sequential conversion of acetyl-CoA) to butyrate is catalyzed by the enzymes phosphate butyltransferase and butyl kinase.6
5.1. Acid production pathways
Clostridia grow exponentially in the first phase of fermentation (acidogenesis phase) along with the formation of acids (acetate and butyrate), leading to a decrease in the pH to 4.5.111 In the acidogenic phase, the glycolysis pathway is active to produce pyruvate consuming glucose, which is further converted to acetyl-CoA (Fig. 1).111,112 Acetyl-CoA is the main precursor for the synthesis of acetate, butyrate, ethanol, butanol and acetone anaerobically (Fig. 1). Acetate and butyrate are produced in the acid producing phase through two analogous steps from acetyl-CoA and butyryl-CoA respectively. Phosphate acetyltransferase and acetate kinase are the enzymes involved in acetate formation, while phosphate butyltransferase and butyrate kinase are active for butyrate synthesis. Four enzymes, namely thiolase, 3-hydroxybutyryl-CoA dehydrogenase, crotonase, and butyryl-CoA dehydrogenase catalyze the synthesis of butyryl-CoA from acetyl-CoA.23,113 The acids produced during the acidogenic phase can permeate through the cell membrane and are involved in triggering of solventogenic phase.
5.2. Solvent production pathways
The acid production slows down due to the low pH during the final stage of acidogenesis. To compensate for the unfavorable effect of low pH, the organism shifts its metabolic activity from the acidogenesis phase to solventogenesis phase. In this phase, acetate and butyrate are consumed as substrates for the biosynthesis of acetone and butanol while no growth is observed.112 However, the metabolic activity of this complex phase shift is different for different organisms. For example, conversion of butyrate to butanol is hardly observed in the case of C. saccharoperbutylacetonicum N1-4 in the absence of glucose in the culture medium. The highest butanol yield (0.671 mol butanol/mol butyrate) from butyrate was observed in presence of culture medium glucose.114 Acetyl-CoA and butyryl-CoA are the key intermediates in synthesizing ethanol and butanol (Fig. 1). These pathways produce acetaldehyde and butyraldehyde respectively, in the presence of two sets of dehydrogenases. The reduction of butyryl-CoA to butanol is mediated by butyraldehyde dehydrogenase and butanol dehydrogenase.111,115 Six enzymes (thiolase, 3-hydroxybutyryl-CoA dehydrogenase, crotonase, butyryl-CoA dehydrogenase, butyraldehyde dehydrogenase, and butanol dehydrogenase) and the corresponding seven genes (thiL, hbd, crt, bcd, etfA, etfB, and adhe) were identified for the conversion of acetyl-CoA to butanol (Fig. 1).115–118 The activity of butanol dehydrogenase in both C. acetobutylicum and C. beijerinckii, was NADPH dependent instead of NADH.119 Ethanol could be produced independently under certain adverse medium conditions. However, ethanol formation was observed in a small amount in comparison to acetone and butanol. The uptake of acids (butyric and acetic acids) was coupled with the formation of acetone and butanol resulting from the complex mechanism of metabolic pathways (Fig. 1).23,104,111,120 The switchover from acidogenesis to solventogenesis occurs at reduced pH. Bahl et al.121 have suggested that the switchover is an adaptive response of the cells to the low pH of the medium, whereas Ballongue et al.122,123 have suggested that acids produced in the acidogenic phase act as inducers for the biosynthesis of solventogenic enzymes.
6. Cocultures
Co-cultures of the Clostridium strain with enzyme producing microorganisms to simultaneously hydrolyze the cellulose and hemicellulose have been attempted to reduce the cost of pretreatment (hydrolysis) and purification of the hydrolysate. This process is commonly referred to as simultaneous saccharification and fermentation (SSF). Soni et al.124 used mixed cultures of cellulolytic fungi T. reesei and A. wentii to hydrolyze agricultural wastes (bagasse, rice straw, and wheat straw). C. saccharoperbutylacetonicum and/or C. acetobutylicum fermented these wastes to yield 16 g l−1 and 17.3 g l−1 of butanol, respectively. Petitdemange et al.125,126 and Fond et al.127 tried to use mesophilic cocultures of cellulolytic (C. cellulolyticum H10) and glycolytic (C. acetobutylicum) clostridial strains for the direct fermentation of cellulose. However, the major fermentation product was acid (butyric acid), with a small amount of acetone, ethanol, and butanol. A two-stage fermentation process with a co-culture of C. butyricum and C. pasteurianum in the first stage and C. beijerincki and C. pasteurianum in the second stage produced 20% more butanol. Consequently, the high level of butyric acid production in the first stage was effectively reduced to butanol in the second stage. Further, continuous immobilized cultures of C. tyrobutyricum and C. acetobutylicum with corn as a substrate, resulted in a productivity of 4.64 g l−1 h−1 and yield of 42%. C. tyrobutyricum increases the production of hydrogen and butyric acid, whereas C. acetobutylicum effectively converts butyric acid to butanol. This in turn eliminates the products such as acetic, lactic, and propionic acids.128 Qureshi et al.36 used Clostridium beijerinckii P260 to produce ABE from wheat straw hydrolysate in the fed-batch reactor. They found that the simultaneous hydrolysis of wheat straw and fermentation is possible with 100% hydrolysis to simple sugars. Fed-batch fermentation with C. beijerinckii P260 was continued to use glucose, xylose, arabinose, galactose and mannose; obtained from the wheat straw. The culture utilized all the sugar components improving the ABE productivity by 16%.
7. Commercial production and challenges in ABE fermentation
Advanced fuels, (non-ethanol fuels) are high energy and high performance biofuels that include higher molecular weight alcohols (n-butanol and isobutanol) as well as infrastructure compatible hydrocarbons produced from either thermochemical (Fischer–Tropsch fuels) or biochemical (fermentation-derived hydrocarbons) pathways. Biobutanol is likely to be the first of these biofuels to reach the global market.129 One among several partnerships active in this area is the collaboration between BP and DuPont.130 Several other companies are active in this stream, including EEI (D. Ramey), Cobalt, Gevo, and Tetravitae.
During 1950s the ABE fermentation process was not competitive with natural petroleum fuels and hence found to be ignored in the US and Europe. However, some production processes via fermentation continued in China, Russia and South Africa until the early 1980s.12,13,131 China leads efforts to re-commercialize the ABE fermentation process. Over $200 million has recently been invested in China to install 0.21 million tons per annum of solvent capacity with plans to expand it to 1 million tons per annum. There are six major plants that produce about 30
000 tons butanol per annum from corn starch.14 Most plants operate in a semi continuous mode where one fermentation cycle can take almost 21 days. Most of the bio-butanol plants are located immediately next to the ethanol plants to reduce utility and operating costs. Co-located operations tend to share effluent treatment facilities based on anaerobic digestion (AD). Biogas, produced from the AD process, can be used to generate power and heat. The recovery of hydrogen from the fermentation exhaust gas (typically 1/10 of mass of butanol produced) gains an additional value to the process. However, some serious problems are associated with industrial ABE production. In general, there is an extensive need for cheaper feedstocks, improved fermentation performance and more sustainable process operations for solvent recovery and water recycling. Feedstocks are known to contribute most to the production cost. The production cost and profitability of the plant is extremely sensitive to the price fluctuation of the feedstock. The fermentation performance can be improved by using chemical mutagenesis, specific genetic manipulation or by combination of both. The technical and related commercial challenges for the conventional ABE fermentation have been extensively reviewed41,42,45,132 and are summarized in Table 4.
Table 4 The challenges and solutions for ABE fermentation251
Challenges |
Solutions |
High feedstock costs increase the operational cost |
Transition towards cheaper and sustainable feedstocks such as agricultural wastes and residues |
Low butanol titres increase the recovery costs, reduce sugar loadings, increase feedstock costs and increase water usage |
Develop improved microbes with improved solvent titres and/or develop in situ product removal techniques to reduce product toxicity effects |
Low volumetric solvent productivities increase capital and operating costs |
Develop continuous fermentation processes that reduce down time and increase volumetric productivity |
Conventional distillation technique for solvent recovery is energy intensive and relatively expensive |
Develop low energy methods for solvent recovery and purification. Recovery can also be improved by improving the solvent titre |
High water usage is not sustainable and increases the cost of effluent treatment. |
Recycle process water back to the fermentation |
7.1. Acid crash in ABE fermentation
The phenomenon known as “acid crash” is an occasional feature of batch fermentations which are performed without any pH control wherein excess of acid production takes place without a significant switch to the solventogenic phase. It is proposed that the acid crash occurs when the acid concentration in the fermentation broth exceeds the maximum tolerable limit, causing cessation of glucose uptake and rapid termination of solventogenesis after the switch has occurred.133 This phenomenon cannot be confused with that of culture degeneration, which is a feature of the strain rather than of particular batch fermentation and which takes place over a longer period of time, particularly in a continuous culture, and is normally associated with genetic change. In this case there is a slow drift from a solventogenic to an acidogenic culture (due to accumulation of mutant organisms), rather than a switch from an acidogenic to a solventogenic culture.99 Cho et al.134 investigated the effect of acetic acid and formic acid on ABE production by solventogenic Clostridia. They found that C. acetobutylicum and C. beijerinckii could tolerate an acid concentration up to 11.7 g l−1. Moreover, C. acetobutylicum was more vulnerable to weak acids such as formic acid than C. beijerinckii. Hence, C. beijerinckii is preferred against C. acetobutylicum for ABE production by some researchers when using lignocellulosic hydrolysate containing acetic and formic acids.
8. Mathematical models
Ranjan and Moholkar6 recently detailed the mathematical models which have been used by various researchers for ABE fermentation. Papoutsakis135 derived the model equation for butyric acid bacteria fermentation. Chauvatcharin et al.136 have further applied the fermentation model of Papoutsakis for analyzing the culture metabolism of acetone–butanol (AB) fermentation. Besides, the research group at the Technical University of Delft (the Netherlands) has developed a series of models for the continuous ABE fermentation by immobilized C. beijerinckii. The salient features of the model were (i) the substrate (glucose) consumption was written in the form of the Herbert–Pirt equation; (ii) Monod kinetics were used to explain the relation between the substrate concentration and the specific growth rate, whereas the effect of product inhibition was based on the maximum limit of butanol concentration; and (iii) the substrate consumption rate was defined in terms of dilution rate, concentration of substrate in feed, and reactor voidage. Categorizing the biomass in the reactor in two locations, viz. within immobilization matrix, and as freely suspended cells, the latter was used to determine the rate of substrate consumption. The effectiveness factor can be determined using generalized Thiele modulus.137,138 A research group at McGill University (Canada) have developed a ‘process kinetics model’ and a ‘physiological state model’ for the AB fermentation. The ‘process kinetics model’ represents the AB process dynamics, while the ‘physiological state model’ elucidates the key process parameters and their influence in the control of solvent biosynthesis. The major culture parameters affecting the solvent biosynthesis were permeability of cellular membrane and number of active sugar transport sites.139–141 The process kinetics model139,140 assumes the batch fermentation is glucose limited, with no process limitation by nitrogen source and takes the cellular RNA content as the basis. The physiological model differs from the process kinetics model taking into account both intracellular and extracellular culture conditions and it also contains transport parameters for solvents on a cellular level. Further, Jarzebski and coworkers142,143 have proposed a kinetic and physiological model for continuous AB fermentation with two assumptions: (i) solventogenesis is triggered by attaining a given threshold concentration of intracellular butyrate, (ii) the un-dissociated form of butyric acid passes easily through the cell membrane. This model suitably predicts the formation of acids in the case of strong substrate limitations and also the drop in solvent concentration due to increased dilution rate. The model satisfactorily predicts the performance of a continuous as well as a batch fermentation.144–146 Researchers at Nagoya University (Japan) have developed a general framework for an extractive fermentation, which could be applied to batch, repeated batch, and repeated fed-batch modes.147 The model comprised a set of differential equations for biomass formation, substrate utilization and products concentration. Furthermore, Shinto et al.148,149 have developed a kinetic model for ABE fermentation on the basis of the metabolic pathway of C. acetobutylicum ATCC 824.
The solvents produced in the fermentation are extracted by oleyl alcohol and can be recovered by distillation. However, the distillation of the solvents under vacuum at a mild temperature is both practical and reasonable. Hence, Shi et al.150 have proposed a flash extractive fermentation system to assess its performance with a mathematical model. This model comprises six mass balance equations for the cells, substrate, product in the solvent phase, product in the aqueous phase, and overall balance. The simulations with the flash extractive model are summarized as: (i) a highly concentrated feed resulted in noticeable improvement in performance; (ii) the solvent distribution rate increases the total productivity but results in the loss of energy utilization efficiency; (iii) the two-vessel flash system does not simultaneously satisfy the requirement of product purity and energy efficiency.
9. Metabolic engineering
As evident from the previous sections, butanol toxicity to the fermenting microorganisms restricts its concentration in the fermentation broth, resulting in low butanol titer and high production and recovery cost from the dilute solutions. Nevertheless, with the progress in modern molecular biology, several genes involved in the biosynthesis of solvents and acids have been cloned and characterized to study the fermentation physiology. Meanwhile, there is a continuing interest in developing more suitable industrial organisms for butanol production. The Clostridia are not genetically controllable, well-characterized, and fast growing when compared with E. coli and Saccharomyces cerevisiae, the current industrial alcohol producers. They are able to tolerate high concentrations of butanol by the same mechanisms by which they tolerate ethanol. Thus, genetic tools are being used to manipulate the metabolism by introducing the genes responsible for butanol production from C. acetobutylicum into the E. coli151–153 or yeast.154 Achievements in genetic engineering of producing strains, reducing butanol toxicity, improving yield, and simplifying downstream processing can contribute to introduce biobutanol fermentation at a commercial level.155
9.1. Genetic engineering
Genetic modification of Clostridium is widely used by inserting some heterologous genes or overexpressing or knocking out/down some relative endogenous genes to improve the butanol production. As discussed earlier, in Clostridium sp. hexoses and pentoses are catabolized to produce butanol (Fig. 1).156–158 Since C. acetobutylicum is the first choice Clostridium species for industrial biobutanol production, most genetic and biochemical studies focus on C. acetobutylicum as a representative species.
Genetic modification in butanol producing bacteria was attempted after recognizing the butanol and acetone producing genes. Metabolic engineering provides the targeted gene for manipulation prior to the genetic engineering. The TargeTron technology has been utilized to disrupt the acetoacetate decarboxylase gene (adc), which was responsible for acetone production in a hyper-butanol producing industrial stain. This enhanced the butanol ratio from 70% to 80.05%, while acetone production was reduced to 0.21 g l−1.113 Recombinant DNA technology (RDT) is another striking genetic engineering tool for facilitating the solvent producing ability of microbial strains.159 The use of different host organisms other than clostridial strains for butanol production was encouraged by various researchers because of the genetic complexity of the Clostridia and lack of suitable genetic tools. Heterologous organisms (E. coli and some other organisms) with butanol producing genes of clostridia have been constructed45 as discussed in the subsequent sections. Reconstructed microorganism exemplified a butanol and propanol titer of approximately 2 g l−1 with 1
:
1 ratio.160
9.2. Genetic modification of enzymes involved in the biosynthetic pathway
For butyrate formation, phosphate butyryltransferase (PTB) and butyrate kinase (BK) are essential (Fig. 1, Table 5). PTB catalyzes the interconversion of butyryl-CoA and butyryl-P and enables the conversion of butyryl-CoA to butyrate together with butyrate kinase. This enzyme has eight subunits of equal molecular weight.161 Butyryl-P is formed with an optimized pH value of 8.1 which is also optimal for PTB in the physiological path, while in the reverse direction (butyryl-CoA forming), the enzyme is less sensitive and shows a broad pH optimum ranging from 7.5 to 8.7. BK catalyzes the reaction by which butyryl-P is converted to butyrate with concomitant ADP phosphorylation and the reverse reaction by which butyrate is converted to butyryl-P uses ATP as the phosphate donor.162
Table 5 The list of key enzymes of the butanol synthetic pathway in C. acetobutylicum116
Pathway |
Key enzyme |
Abbr. |
Gene |
EC no. |
M
r (kDa) |
Lactate synthetic pathway |
Phosphate acetyltransferase |
PTA |
pta |
2.3.1.8 |
36.2 |
Acetate kinase |
AK |
ack |
2.7.2.1 |
44.3 |
Butyrate synthetic pathway |
Phosphate butyryltransferase |
PTB |
ptb |
2.3.1.19 |
264 |
Butyrate kinase |
BK |
buk |
2.7.2.7 |
85 |
Butanol synthetic pathway |
Acetyl-CoA acetyltransferase |
THL |
thiL |
2.3.1.9 |
41 |
β-Hydroxybutyryl-CoA dehydrogenase |
BHBD |
hbd |
1.1.1.35 |
30.5 |
Enoyl-CoA hydratase (crotonase) |
CRT |
crt |
4.2.1.17 |
158 |
Butyryl-CoA dehydrogenase |
BCD |
bcdetfBetfA |
1.3.99.2 |
33 |
Butyraldehyde dehydrogenase |
BAD |
aad |
1.2.1.57 |
56 |
Butanol dehydrogenase |
BDH |
bdh AB |
1.1.1.1 |
42 |
Acetone synthetic pathway |
Acetoacetate decarboxylase |
AADC |
adc |
4.1.1.4 |
28 |
CoA-transferase |
CoAT |
ctfA/B |
2.8.3.9 |
93 |
Ethanol synthetic pathway |
Acetaldehyde dehydrogenase |
ALDH |
aad |
1.2.1.10 |
96 |
NAD(P)H alcohol dehydrogenase |
ADH |
adh |
1.1.1.2 |
44 |
In the butanol synthetic pathway, six enzymes, including thiolase, are needed to complete the conversion of acetyl-CoA to butanol (refer to Fig. 1; Table 5). Thiolase plays a key role in the production of both acids and solvents, as it efficiently controls the flow of carbon into these pathways. It catalyzes the condensation of two molecules of acetyl-CoA into one acetoacetyl-CoA, which later on is converted to acetone, butyrate or butanol. Regulation of thiolase activity is important for maintaining the C2
:
C4 acid ratio, which has a profound effect on the energy control of the cell. THL may play an indirect role in acid uptake.163 Butyraldehyde dehydrogenase, which is NADH-dependent, catalyzes the conversion of butyryl-CoA to butyraldehyde, a key intermediate in the formation of butanol, accompanied by the oxidation of NAD(P)H and the release of CoA. Butanol dehydrogenase catalyzes the final step in butanol synthesis with the reduction of butyraldehyde to butanol at the expense of a reduced NAD(P). The butanol dehydrogenase from C. acetobutylicum ATCC 824 has two different coenzyme requirements: the NADH-dependent butanol dehydrogenase (NADH–BDH) with higher activity at lower pHs and the NADPH-dependent with higher activity at basic pHs. BCD catalyzes the α,β desaturation of acyl-CoA substrates.164 Enzymes such as PTB, BK, PTA and AK are more active in the acidogenic phase resulting in the production of only acids (butyric and acetic). The activities of these enzymes are known to be decreased by the accumulation of the acids. Meanwhile, enzymes such as CoA, THL and BDH in the solventogenic phase begin to be active and the pH value stabilizes or increases slightly because most of the acids are taken up and converted into solvents (butanol, acetone and ethanol).
Mermelstein et al.165 constructed a recombinant C. acetobutylicum strain with a pFNK6 plasmid, which contains an ace operon including adc and ctfA/B genes. Compared to the control strain, the final concentrations of acetone, butanol and ethanol were increased by 95%, 37% and 90%, respectively. Harris et al.166 constructed a stain, PJC4BK, in which buk was inactivated. When the pH was ≥5, the strain appeared to be a super solvent producer yielding 16.7 g l−1 of butanol, 4.4 g l−1 of acetone and 2.6 g l−1 of ethanol. Butyrate levels were low throughout all fermentations and never exceeded 20 mM. Tummala et al.167 downregulated genes coding acetoacetate decarboxylase and coenzyme A transferase with an antisense RNA. Further, they attempted to increase the butanol
:
acetone ratio through overexpression of the alcohol–aldehyde dehydrogenase gene (aad) and downregulation of CoA transferase with an antisense RNA against ctfB (the second CoA gene on the polycistronic aad–ctfA–ctfB). Jiang et al.113 disrupted the acetoacetate decarboxylase gene (adc) in C. acetobutylicum EA 2018 to increase the share of butanol by eliminating the production of other by-products, such as acetone. They found that the butanol
:
acetone ratio was improved from 70% to 80.05%, and the acetone production was reduced to approximately 0.21 g l−1 in the adc-disrupted mutant.
9.3. Genetic modification to increase the solvent tolerance of the strain
During the last few years, volumetric productivity has been greatly increased by applying improved physiological and biological methods. However, when the solvent concentration reaches 20 g l−1, the clostridial cellular metabolism ceases and the butanol production also impedes the microbial growth. This difficulty to achieve more concentrated solvent production with the wild type strain can be overcome by alternative modified microorganisms which tolerate higher amounts of solvent. Hence, developing a tolerant strain is more feasible by genetic modification.168,169 Alsaker et al.170 revealed that overexpression of spo0A (multifunctional regulator) by the DNA microarray technique increased the tolerance and prolonged the metabolism in response to butanol stress. Tomas et al.171 also constructed an engineered strain with overexpressed spo0A which exhibited butanol production higher than with a spo0A inactivated strain. Although the solvent tolerance mechanism is still unclear, several genes involved in it are being studied by various researchers. Borden and Papoutsakis172 built the strain 824(pCAC0003) and strain 824(pCAC1869), by inserting CAC0003 and CAC1869 genes which exhibited 13% and 81% increases in butanol tolerance respectively when compared with the plasmid control strain. A butanol-tolerant mutant strain of C. beijerinckii NCIMB 8052 was constructed by using an antisense RNA targeting the gldA gene which encodes glycerol dehydrogenase. The overexpression of some non-enzyme proteins such as GroEL, a class I heat-shock protein, can also enhance the solvent tolerance.169,173 The improvement in the solvent tolerance to increase the final solvent concentration is presumably because of stabilization of solventogenic enzymes.
9.4. Genetic modification to control the sporulation
Solvent accumulation to the level at which it hampers cell growth may stimulate the bacteria to produce the spores. Sporulation allows the active cells to enter into a dormant state wherein they lose the ability to produce solvents. Hence, additional efforts on non-sporulation or delayed sporulation are being continued by various researchers. spo0A also has been shown to affect the expression of genes at the transition from the acidogenic phase to the solventogenic phase in solventogenic Clostridia.174 It controls the beginning of sporulation, the development of competence for DNA uptake and many other stationary phase-associated processes.174 abrB, was described as an inhibitor of sporulation which acts in the opposite way to the spo0A, and acts as both a positive and a negative regulator of cell competence at various stages in the life cycle and regulates the catabolite repression in carbon-limited media. Scotcher et al.175 constructed C. acetobutylicum ATCC 824 (pMSpo) via antisense RNA targeted technology which prolonged the solventogenesis and increased the production of ethanol (225%), acetone (43%) and butanol (110%) when compared with a control.
9.5. Phase transition regulation from acidogenesis to solventogenesis
A better understanding of acidogenesis and solventogenesis phases along with the phase transition is important when performing systems-level metabolic engineering. The gene expression analyses of C. acetobutylicum176,177 and C. beijerinckii,150 revealed that during the acidogenic phase, a two fold increase in the expression of the pta and ack genes responsible for acetate formation was observed. Likewise, the expression of ptb and buk genes responsible for butyrate formation were up-regulated in both the clostridial strains during the acidogenic phase with varied expression levels in C. acetobutylicum (1.5- to 2.3-fold)176 and C. beijerinckii (2-fold).150 A transcriptome analysis for the evaluation of gene expression levels during phase transition in C. acetobutylicum with phosphate-limited continuous cultures showed that the expression levels of the adhE1, ctfA–ctfB, and adc genes were induced during the solventogenic phase (pH 4.5) compared with the acidogenic phase (pH 5.8).178 It was also found that, under phosphate-limited continuous culture conditions, the adhE1 and adhE2 genes were antagonistically regulated.178 However, contrary to previous reports,176,177 the expression levels of the thlA, hbd, and crt genes were strongly down-regulated during the transition from the acidogenic phase to the solventogenic phase.178
9.6. Selective butanol production by improved fermentation pathway
Although butanol is the major product of Clostridia, it can also produce acetone and ethanol as associated by-products during the conventional ABE fermentation. To increase the butanol yield and to reduce the recovery costs, high butanol selectivity is desirable.113,179,180C. acetobutylicum M5 strain is a mutant of C. acetobutylicum ATCC 824 and it cannot produce butanol due to the absence of the megaplasmid pSOL1.181C. acetobutylicum M5 was metabolically engineered with an overexpression of adhE1 gene under the control of the ptb promoter to restore the selective butanol production without acetone.115,179 In another study, the butanol selectivity was increased to 0.82 g g−1 ABE by disrupting the adc gene encoding acetoacetate decarboxylase in C. acetobutylicum and regulating electron flow by the addition of methyl viologen into the culture medium.113
9.7. Biosynthetic pathway in heterologous organisms
Due to complex and unknown genetic and physiological characteristics of Clostridia, it is much more difficult to metabolically engineer this strain as compared to the commonly used industrial microorganisms, such as E. coli and S. cerevisiae. Thus, there has been considerable effort to introduce the clostridial fermentative pathway into several heterologous organisms, such as E. coli, Bacillus subtilis, and S. cerevisiae, to possibly overcome some of the problems associated with the clostridial butanol production.
Atsumi et al.153 reported the reconstruction of the functional butanol biosynthetic pathway in E. coli by the introduction of the C. acetobutylicum thl, hbd, crt, bcd, etfAB and adhE2 genes. Further, they identified alternatives for thiolase and butyryl-CoA dehydrogenase, and found that the expression of E. coli atoB instead of C. acetobutylicum thl increased the butanol titer under microaerobic conditions. A further increase in butanol titer was achieved by removing the competing pathways, and finally, a modest maximum of 0.55 g l−1 butanol could be produced by cultivating an engineered E. coli in a nutritionally rich medium containing 20 g l−1 glucose. The potential of S. cerevisiae, B. subtilis, Pseudomonas putida, and Lactobacillus brevis as a butanol-producing host were assessed by various researchers.118,154,182,183 Cloning into P. putida, B. subtilis118 and S. cerevisiae154 produced 0.11 g l−1, 0.024 g l−1 and 0.0025 g l−1 butanol respectively. Transformation of crt, bcd, etfB, etfA, and bcd enabled L. brevis to form 0.3 g l−1 butanol on glucose media, using its own thiolase, aldehyde and adh genes.46,182 These results suggested that the introduction of a clostridial fermentative pathway into a heterologous host does not allow efficient butanol production and requires much more metabolic engineering and optimization efforts. More detailed analyses of metabolic fluxes, energy and redox balance, the expression and activity characteristics of enzymes, and interactions between the host metabolism and heterologous butanol pathways need to be performed. Besides, bacteria other than Clostridia, are not capable of utilizing diverse carbon substrates, including both C5 and C6 sugars, for economical butanol production. The two different strategies to enhance the butanol production in heterologous hosts are (a) to enhance the butanol forming flux by fine-tuning the expression levels or introducing an alternative enzyme that catalyzes the same reaction (b) alternative pathways leading to butanol formation or its key intermediates such as L-threonine, 2-ketobutyrate and 2-ketovalerate to form butanol in E. coli.184
9.8. Metabolic engineering for isopropanol–butanol–ethanol biosynthesis
Clostridium metabolic engineering can also be accomplished to produce a mixture of IBE (2-propanol–butanol–ethanol) solvents instead of standard ABE. This mixture of solvents as such can be sold as chemicals or used to replace gasoline in internal combustion engines. Such genetically modified Clostridium strains could be used for industrial production of “green” chemicals and biofuels from renewable and sustainable resources such as lignocellulosic biomass. Since, C. acetobutylicum does not possess a secondary alcohol dehydrogenase (adh); it is unable to produce 2-propanol (isopropanol), from acetone. In order to modify the organism to produce 2-propanol the adh gene for 2-propanol dehydrogenase from C. beijerinckii NRRL B593 can be inserted into C. acetobutylicum DSM792.185–187 The resulting modified C. acetobutylicum DSM792-pADH1 strain produced up to 14.3 g l−1 IBE solvents from standard glucose media and 5 g l−1 of IBE solvents from hydrolyzed spent liquor188 from spruce chips. C. acetobutylicum PJC4BK (pIPA3-Cm2) lacking the butyrate kinase (buk) gene and containing synthetic acetone operon (adc–ctfA–ctfB) produced 20.4 g l−1 IBE solvents from CGM media supplemented with 80 g l−1 glucose.187
9.9. Mutation
Random mutation has been performed by few researchers to alter the DNA sequence and/or regulation of the genes responsible for ABE production. Lin and Blaschek,189 developed a SA-1 mutant strain from C. acetobutylicum ATCC 824 with serial enrichment of diluted n-butanol. The strain produced higher butanol and lower acetone titers with significantly enhanced butanol tolerance (121% higher) over the native strain. Besides, it also showed a higher carbohydrate utilization rate and higher α-amylase activity to prove its potential in the utilization of waste cellulosic materials as feedstocks. Another mutated strain of C. acetobutylicum (MEMS-7) obtained after treatment with N-methyl-N-nitro-N-nitrosoguanidine and ethyl methane sulphonate and under UV exposure showed better potency in molasses. It was recognized as an effective mutant showing 20% higher butanol yield than the parent strain.190 The most significant achievement with mutation was the development of C. beijerinckii BA101 strain which showed the highest butanol production capacity so far reported (19–20 g l−1).34,36,191,192 To eliminate the tedious and expensive maintenance of anaerobic conditions a successful mutant of E. coli (which can grow aerobically) was recognized for the synthesis of 3-methyl-1-butanol (9.5 g l−1).120,193
9.10. Systems biology
Systems biology is the study of systems of biological components, which may be molecules, cells, organisms or entire species. Understanding complex cellular systems is essential for the construction of new phenotypes through metabolic engineering or synthetic biology. Traditional molecular biology methods are limited to a few metabolic engineering modifications that could be evaluated simultaneously, and hence it was difficult to get a systems perspective. The chimeric pathway from C. acetobutylicum using enzymes CRT and ADHE2 was introduced in the n-butanol pathway, from Ralstonia eutropha and Treponema denticola with PHAA and HBD and TER respectively. The overexpression of aceEF to increase the acetyl-CoA pool, from the metabolic system was evaluated and resulted in a 1500-fold increase in the 1-butanol titer from the initial 3 mg l−1 to 5 g l−1.194,195 NADH reducing pathways, such as, succinate, lactate, ethanol and acetate pathways were blocked by deletion of frdBC, ldhA, adhE and pta, respectively to produce 15 g l−1n-butanol. An implementation of NADH drain in the form of n-butanol pathway composed of enzymes from C. acetobutylicum (HBD, CRT and ADHE2) together with the highly active endogenous acetyl-CoA acyltransferase (atoB) and an irreversible trans-enoyl-CoA reductase (ter) from T. denticola.196
Although S. cerevisae seems to be an ideal n-butanol producing host organism, metabolic simulations suggested the lower butanol and propanol yields with it when compared to E. coli are because of the limited flexibility of the central metabolism.197–199 Industrial production of n-butanol with yeast targets to increase the acetyl-CoA pool by overexpression of the pyruvate dehydrogenase multienzyme complex (lpdA, aceE, aceF) from E. coli.200
10. Extremophiles in biofuel synthesis
Extremophiles refer to microorganisms, generally prokaryotic, which thrive in environmental conditions considered to be hostile to humans.201 Temperature, pH, salinity, pressure, radiation, light and water content are some of the parameters which contribute towards the extreme conditions. Several advantages are associated with the use of extremophiles in industrial applications, in particular during biofuel production. Extremophiles are robust in nature, produce stable enzymes and are often able to tolerate stringent changes in environmental conditions, such as pH and temperature. The majority of extremophiles used in biofuel production are from thermophilic sources, which can tolerate fluctuations in pH, temperature and other environmental changes an attribute which offers a clear advantage in the development of a commercially viable process.201–203 Moreover, thermophilic industrial fermentations are less susceptible to microbial contamination and require lower energy inputs as a result of the reduced cooling steps during the fermentation. Besides, the removal of any volatile products, which in turn minimizes the problem of product inhibition, is facilitated.204
Knowing the fact that most microorganisms are unable to grow at butanol concentrations above 2%; there are certain species of Bacillus and Pseudomonas that are able to tolerate butanol concentrations as high as 2.5–7%.205–207Pseudomonas achieves high solvent tolerance by removal of solvent using efflux pumps and physico-chemical changes in their membrane lipids.206,207P. putida S12 showed moderate tolerance to butanol, while other P. putida strains have been evolved to tolerate 6% w/v butanol.208,209 Hence, the recent engineering of P. putida to produce butanol has been tried by few researchers.118 The UK-based company Green Biologics (http://www.greenbiologics.com) uses a mixture of thermophiles and thermostable enzymes for the production of butanol from waste biomass which is commercially sold as Butafuel™.204 Recently, the citramalate synthase (CimA) from the extremophile Methanococcus jannaschii was found to increase the activity over a temperature range of 30 °C to 70 °C and overexpressed in E. coli for the production of both n-propanol and n-butanol.151,152,210 The citramalate pathway directly converts pyruvate to 2-ketobutyrate bypassing threonine synthesis. The biotechnology company Gevo™ (http:\\www.gevo.com) adopted this technology to use E. coli for butanol production.
11. Solvent recovery techniques
Though many attempts have been made to improve butanol production, the concentrations of butanol in the fermentation broth are not yet satisfactory. The recovery of low concentrations of butanol by traditional distillation is energy intensive and hence economically not feasible.45 For every 1 ton of solvent, approximately 12 tons of steam is required. Although improvements can be made to the conventional distillation processes, nonconventional methods are required to significantly reduce the energy and associated costs. Therefore, simultaneous fermentation and product removal techniques have been developed to reduce the cost of butanol recovery.41,96,211–214 Some butanol recovery techniques are discussed in subsequent sections.
11.1. Adsorption
Use of adsorbents that have high affinity for the removal of solvents from the fermentation broth is a feasible technique for in situ product recovery. The adsorbents have the advantages of ease of separation, nontoxicity and ease of regeneration/reuse. In this technique, butanol is first adsorbed by adsorbents from the fermentation broth and further desorbed by heat treatment or displacers to yield concentrated butanol solutions. Activated carbon, silicalite, polymeric adsorbents such as polystyrene cross-linked resins, zeolite, and molecular sieves are the few among numerous alcohol selective adsorbents. However, silicalites are the most favoured adsorbents which possess zeolite-like structure and hydrophobic properties that can selectively adsorb small organic molecules like C1–C5 alcohols from dilute aqueous solutions.41,215 Coupling of adsorptive removal of solvents with a membrane filtration–centrifugal system overcomes the limitation of biofilm formation by cells from the fermentation broth. The energy requirement for butanol recovery by adsorption–desorption was calculated to be 1948 kcal kg−1 butanol, which is significantly smaller than the energy requirement for other techniques such as steam stripping distillation (5789 kcal kg−1 butanol), gas stripping (5220 kcal kg−1 butanol), and pervaporation (3295 kcal kg−1 butanol).
Other adsorbents, such as activated carbon and polymeric resins, suffer from the fouling by cells and other media components with adsorption of butyric and acetic acids, to reduce the solvent yield.216 The modifications in adsorbent characteristics, such as increasing hydrophobicity and selectivity, are possible means of improving the feasibility of adsorptive recovery of butanol which overcomes the larger scale operation limitations.216–218
11.2. Liquid–liquid extraction
The removal of butanol or ABE from the fermentation broth by liquid–liquid extraction is an important technique. In this method, the fermentation broth is continuously contacted with a water-immiscible extractant that selectively extracts ABE from the broth, leaving all other components, such as nutrients and cell, in the broth. The choice of extractant is the most crucial aspect of an integrated liquid–liquid extraction fermentation process. The extractant used for the fermentation process should not be toxic to microorganisms and should have high selectivity for the ABE solvents (highest in particular for butanol). It also must have nearly zero partition coefficients for nutrients, substrates, and intermediates of fermentation. Unfortunately, the liquid–liquid extractants with high butanol distribution coefficients are toxic to the culture, while nontoxic liquid extractants have low butanol distribution coefficients. Evans and Wang,96 used mixed extractants to balance both the drawbacks. The mixed extractant that contained 20% decanol (high partition coefficient to butanol, but toxic) in oleyl alcohol (low partition coefficient to butanol, but non-toxic) enhanced butanol production by 72% under pH-controlled conditions.96,79 This mixed extractant further reduced the viscosity when compared with the viscosity of single extractant. Some common toxic solvents used for blending with oleyl alcohol (highly viscous) are kerosene, benzyl benzoate, and decane.219 A number of researchers220–222 have reported the removal of butyric acid with extractants. The saturation of extractant with butyric acid prior to contacting with broth or to add supplemental acid directly to the broth, did not affect the butyric acid extraction. In addition, the extractant is also expected to have some advantageous features such as high interfacial tension with water that would assist its easy separation, low viscosity, negligible water solubility, high thermal stability, easy regeneration, biodegradability and high density difference, which would assist counter current operation. However, several problems are associated with liquid–liquid extraction, such as toxicity to the cells, the formation of an emulsion, loss of extraction solvent, and the accumulation of microbial cells at the extractant and fermentation broth interphase. The ABE solvents are recovered from the extractant using distillation.6
11.3. Pervaporation
Pervaporation allows the selective removal of volatile compounds from the fermentation broth using a membrane. This membrane is selectively permeable to the solvents in the fermentation broth. Thus, when contacted with the fermentation broth, the ABE solvents in the broth first get solubilized into the membrane, followed by diffusion through the membrane and evaporation on the other side (or permeate side) at low pressure. These vapors can be recovered by either condensation or by using a sweep gas that removes the vapors. In this process, a phase change occurs from liquid to vapor. Concentration and vapor pressure gradient is used to allow one component to preferentially permeate across the membrane.89 This mechanism by which volatile solvents are removed from the fermentation broth is known as the solution–diffusion mechanism. As it is a selective removal process, the desired component requires a heat of vaporization at the feed temperature. The heat required for evaporation of solvents is taken from the broth which reduces the temperature of the broth. The efficacy of pervaporation is measured by two parameters: the selectivity (a measure of the selective removal of volatiles) and flux (the rate at which an organic/volatile passes through the membrane per m2 membrane area).41 The solvent selective (asymmetric) membranes available in the market are made up of poly (dimethyl siloxane), poly (methoxy siloxane), polytetrafluoroethylene, and silicone rubber as the skin of the membrane with supports made of polysulfone or polyacrylonitrile.
Advantages of pervaporation include low energy consumption, entrainer is not required and low contamination frequency. However, the membrane needs to be regenerated at some point, because a “swelling” effect makes the membrane more permeable but less selective when material passes through the membrane.45
Ranjan and Moholkar6 detailed the features of overall mass transfer through the membrane as (a) the mass flux through the membrane is inversely proportional to the thickness of the membrane. (b) The resistance of mass transfer in the liquid phase is determined by the flow rate and viscosity of the medium and (c) the resistance to mass transfer is governed by the pressure drop which leads to the reduction in concentration gradient and also the flux. In addition to these, the temperature of the fermentation broth is also a critical factor governing flux through the membrane. Every 10 °C rise in the temperature of the fermentation broth doubles the membrane flux.
11.4. Perstraction
The perstraction operation essentially combines principles of pervaporation and liquid–liquid extraction in that it is a solvent extraction process combined with membrane extraction. The membrane contactor (preferably in the form of hollow fibers) provides a surface area where the two immiscible phases (fermentation broth and the extractant) can exchange butanol. Since there is no direct contact between the two phases this process overcomes several pitfalls of the liquid–liquid extraction process such as solvent toxicity, emulsification, and cell aggregation at the liquid–liquid interface. Besides, an independent control over the flow rate of broth and extractant is also advantageous. In this system, butanol diffuses preferentially across the membrane, while other components and fermentation intermediates (acetic and butyric acids) are retained in the aqueous phase.223 An arrangement of loops of hollow fiber membranes in series for operation in the counter current mode gives a true plug flow character to both phases and an effective removal of butanol. Overall mass transfer in this case is determined by the individual mass transfer coefficients on the aqueous (fermentation broth) and organic (extractant) side as well as the mass transfer coefficient of the membrane. Although the mass transfer coefficient of the membrane is difficult to estimate, typical values of ∼10−7 m s−1 can be assumed. The overall resistance to mass transfer is mostly contributed by the membrane itself (∼86%). The increased diameter of the hollow fiber membrane, with concurrent rise in the volumetric flow rate reduces the mass transfer resistance which maintains the constant velocities through the fiber.224 The membrane does, however, present a physical barrier that can limit the rate of butanol extraction.41
11.5. Liquid membranes
A promising solution to large mass transfer resistance offered by dense polymeric membranes is in terms of liquid membranes. This process requires just a small amount of solvent to cover the support membrane. The fluidity of the organic film leads to the higher diffusion coefficient and high fluxes of solvent. However, the stability of the film is the major problem. Matsumura and Kataoka225 have reported pervaporation through a liquid membrane supported with a hydrophobic microporous polypropylene flat sheet wherein the stability of the membrane was found to depend on the surface tension of feed solution. The principle components of the liquid membranes were oleyl alcohol, di-n-butyl phthalate, and tricresyl phosphate.
11.6. Gas stripping
The gas stripping technique involves removal of ABE solvents in the gas phase, followed by recovery of solvents through condensation. Gas stripping allows the selective removal of solvents from the fermentation broth and uses no membranes which suffer from fouling and clogging. Gas stripping can be applied for in situ butanol recovery during the ABE fermentation.226–228 The production of ABE is accompanied by the generation of gases (CO2 and H2) which are being used to recover butanol during simultaneous fermentation and in situ recovery to make the process simpler and more economical.227,228 The process is directed by vapor–liquid equilibrium. Oxygen-free nitrogen can also be sparged through the fermentation broth and subsequently cooled in a condenser. As the gas is bubbled through the fermentor, it captures the ABE which is further condensed and collected in a receiver vessel. The gas recovered after condensation can be recycled back for further cycles. A separate stripper can be used in some cases to strip off solvents and the stripper effluent can be then recycled back to the reactor.226 Ezeji et al.71 investigated the production of butanol in an integrated fed-batch fermentation-gas stripping product-recovery system using C. beijerinckii BA101, with H2 and CO2 as the carrier gases. They employed a 500 g l−1 concentrated glucose solution during fermentation, and achieved 13-fold more solvents (232.8 g l−1) and fourfold higher productivity (1.16 g l−1 h−1) in this integrated system than in the non-integrated system. Apart from simplicity of this process, other advantages such as clean volatile products, use of in situ gas for stripping and no mass transfer resistance makes it most suitable for application on a large scale.6
12. Energy consumption and wastewater generation
The energy consumption of the process is a vital criteria when choosing the recovery technology in addition to simple operation, scalability, and cost. The recovery technologies (gas stripping, flash fermentation, vacuum fermentation, pervaporation) presumed the butanol concentrate to be in the aqueous phase, which is compatible with the distillation system. Although the continuous fermentations have the indisputable advantage of enhanced productivity, these fermentation systems, when not accompanied by integrated product recovery, have the worst environmental performance in terms of wastewater footprint.229 Pervaporation is the most favored technology as compared to other purification techniques according to the distillation duty and wastewater footprint criteria. The increase in butanol concentration from 12.81 to 105.35 g l−1 in the product stream with the reduction in the energy consumption of the distillation system of 65.8% (from 24.6 to 8.4 MJ kg−1 butanol) was accompanied by a substantial decrease in the wastewater footprint.230 For pervaporation and gas stripping, the total energies (integrated product recovery technology and distillation) calculated by Qureshi et al.231 are 13.8 and 21.8 MJ kg−1 butanol, respectively. These values support the analysis that pervaporation is very attractive from an energy efficiency viewpoint.229
13. Economics of biobutanol fermentation
The mass and energy yields determine the absolute, theoretical limits to the process economics. The theoretical mass and energy yield of ABE fermentation are 37% and 94% respectively, calculated on the basis of product ratio and energy combustions in the fermentation.214 The substrate costs, accounting for approximately 60% of the total production cost, play a major role in the economics of ABE fermentation.232,233 In order to reduce substrate costs in typical bulk-chemical fermentation processes,234 the fermentation plant must be able to use a variety of substrates including those of low grade substrates such as lignocellulosic biomass, waste material from food and feed industries and waste from agricultural industries. Qureshi and Blaschek235 published ABE butanol production costs at $1.56 gal−1 based on $1.80 per bushel corn feedstock cost. If the feedstock is raised to $3.35 per bushel, their estimated butanol cost would be $2.10 gal−1. Although the economy of ABE fermentation is more sensitive to the price of the substrate than yield; it is estimated that the butanol fermentation process will not be feasible if the yields are less than 25% w/w.214 Strain improvement is an effective technique to improve the yield; however it has a lower influence on the process economics as compared to other factors (mainly cost of substrate and product recovery costs). Interestingly, a capital cost of butanol fermentation was higher and production costs were less than the petrochemical production of butanol.236 Batch fermentation processes have been found less economical than continuous processes as they demand additional sterilization steps for vessels, pipes and valves. However, the contamination problem in continuous fermentation is a crucial challenge. The product market is another significant parameter in the economics of butanol fermentation. The present sizeable market of ABE fermentation is still fascinating industrialists and researchers and it is expected that after adapting to biobutanol as a liquid fuel, the market demands will be high. By-products including acetone, ethanol, H2, and CO2 can also contribute significantly to the butanol production economics.235 It should be noted that butanol production itself is a cost-intensive methodology and recovery technology after the fermentation results in a higher cost-intensive process. Improvement in butanol tolerance of strain and selective in situ solvent removal can enhance the fermentation time, productivity and other economic feasibility aspects of the process80,237 which can be materialized in the economic feasibility of ABE fermentation.
Investment costs largely affect the economic assessment of the ABE fermentation process. The large capital investment before the actual production starts has a huge impact on the overall economics of the process. Besides, investment costs directly increase the total costs both as depreciation and for financing (interest and repayments). Hence, major reductions in the investment costs and/or delaying investments especially until the production starts will help to improve the process economics. Aspen Plus simulations have been used to obtain the material and energy balance data, size the specified equipment and to calculate cost structure. It was also used to calculate the fixed (labor and supplies) and variable (raw materials) operating costs of the plant.
The overall production cost data for non-existing or non-commercial biofuel processes (bioethanol, biobutanol) are less readily available and largely detailed with techno-economic models and evaluations. An identical economic assumption reveals that, the product yield on substrate of corn ethanol is almost twice compared to corn butanol. However acetone, ethanol, and hydrogen co-products in corn butanol process contribute more significantly to the net production cost as compared to the corn ethanol process. This result in an overall production cost of butanol to be $1.96 gal−1 compared with $1.53 gal−1 of ethanol produced from corn.238 Besides, the total project investment (TPI) is also doubled for the corn butanol process as compared to the corn ethanol. This is because of the low feedstock yield, and a more complicated separation of biobutanol mixtures and water from the fermentation beer with low solvent concentrations.
Marlatt and Datta236 have reported that with an improved strain tolerance for higher butanol concentrations and an increase in volumetric productivity by about 50%, the production costs for biobutanol would be similar to the production costs for synthetic butanol. Moreover, Woods239 found that if the final solvent concentration can be increased by up to 22–28 g l−1 and if the fermentation time of the batch fermentation of 40–60 hours can be maintained, the ABE fermentation should be industrially feasible. In order to reach economic viability, reductions in both conversion cost-intensity and in recovery cost-intensity were required. The process economics can further be increased with in situ product removal techniques.79 The use of the two-stage continuous solvent-producing cultures with the immobilized biomass or biomass-retention achieves higher solvent productivities with improved substrate consumption and reduced the solvent toxicity problem.80
14. Derivative markets for n-butanol
The US consumption of butanol is 740
000 metric tons per year, which, at the current price of about $0.90 per pound, represents a $1.47B market. The predicted annual growth rate for butanol in the USA is 2.2%. Global consumption of butanol is in the order of 2.9 million metric tons, a $5.7 billion market, with a predicted annual growth of 4.7%. The market for n-butanol is more than 10 times that of the isobutanol, for which the US and global consumption is reported to be 42
000 and 509
000 metric tons, respectively.240,241 Recently, Mascal,242 reviewed the technical description of the range of commercial products accessible from n-butanol, derived from renewable sources (biobutanol). Primary derivatives of n-butanol in which the oxygen functionality of the molecule is retained (butyraldehyde and butyric acid) and n-butanol itself have mainstream applications in the solvent, polymer, fuel oxygenate, and/or specialty chemical markets. The butanol dehydration products (butenes and butadiene) present additional opportunities in the hydrocarbon fuel and synthetic elastomers markets (Fig. 2).
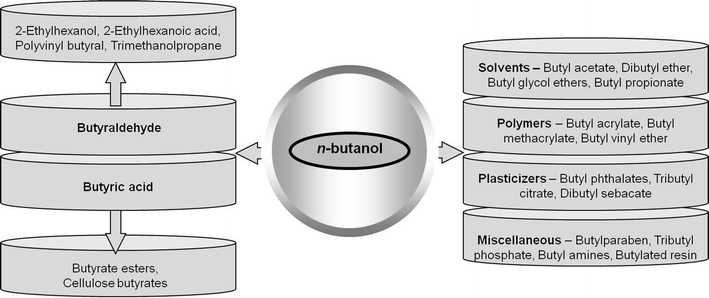 |
| Fig. 2 Derivative markets for butanol.242 | |
15. Conclusions
Since the 19th century, many investigators have noted the applicability of biobutanol as an advanced biofuel in several industries. Butanol from biomass is a better renewable fuel for replacing gasoline than ethanol. As evident from the foregoing review biobutanol is in the process of its commercialization and studies on the development of further economic process are continuing rapidly. None of the starch and sugar containing crops can make fermentation economically feasible on the basis of the current market needs and scenario. Consequently, cheaper agricultural wastes (lignocellulosic materials) and other industrial wastes may be suitable for the economical ABE fermentation. The feedstock cost comprises a very large fraction of the overall production cost, and hence selection of feedstock is an important parameter that needs to be taken into consideration for the process economy.
The complex metabolism of C. acetobutylicum has been elaborated from different perspectives. Studies regarding solvent, particularly butanol production in C. acetobutylicum focused on different aspects such as the development of continuous fermentation technology, improvements in strain tolerance towards butanol, understanding the physiological relationships between acidogenic and solventogenic phases, and genetic modifications. In spite of several attempts in improving the industrial strains by mutation and genetic manipulations, none of the efforts were successful. However, efforts are still needed in identifying better butanol tolerance strains. Development of novel strains may be more challenging as butanol has a direct effect on the cell membrane ceasing molecular activity of the cell. Despite substantial progress in the development of strains for the production of advanced biofuels there is still a need for further improvements to meet the industrial requirements in terms of yield, titer and productivity. Thus, further engineering is required, not only to improve the yield and productivity, but also other important parameters such as tolerance towards the product of interest and the ability to use complex feedstocks from biomass. Simultaneously, recombinant DNA technology in non-clostridial organisms was unable to improve the yields over native clostridial bacteria. Hence, focus should now be diverted on developing more suitable genetic tools for the better polycistronic expression in the host microorganism. Much improvement is still needed to make a biobutanol process economically competitive. However, it is likely to become a competitive process by systems-level metabolic engineering of strains based on the recent availability of complete genome sequences and new metabolic engineering tools for Clostridia. An optimal bioprocess for butanol production can be developed by integrating the fermentation and downstream processes with strain development. The development of process technologies with efficient butanol recovery techniques may be easily achievable and crucial in economics. New recovery processes such as gas stripping and advanced membrane separation may contribute to the economic feasibility in future. Multidirectional endeavors are constrained in achieving the economical usefulness of microbiological butanol synthesis, which will be competitive with petrochemical based butanol. Most of the literature reports on various facets of ABE fermentation are still on laboratory scale, with very few studies on bench or pilot scale. There is an urgent need to assess viability of the process designs on economical pilot scale. One of the biggest challenges in the future will be the scaling-up process in a cost-effective manner.
References
- K. S. Malhotra and N. M. Nahar, Int. J. Energy Res., 1982, 6(3), 283–292 CrossRef
.
- P. Kanniappan and T. Ramachandran, Int. J. Energy Res., 1998, 22(13), 1121–1132 CrossRef
.
- V.-N. Hoang and M. Alauddin, Int. J. Energy Res., 2011, 35(6), 459–476 CrossRef
.
- J. M. Jones, L. I. Darvell, T. G. Bridgeman, M. Pourkashanian and A. Williams, Proc. Combust. Inst., 2007, 31, 1955–1963 CrossRef PubMed
.
- R. Kikuchi, R. Gerardo and S. M. Santos, Int. J. Energy Res., 2009, 33(2), 186–193 CrossRef
.
- A. Ranjan and V. S. Moholkar, Int. J. Energy Res., 2012, 36, 277–323 CrossRef CAS
.
- F. N. Alasfour, Appl. Therm. Eng., 1997, 17(6), 537–549 CrossRef CAS
.
- S. M. Lee, M. O. Cho, C. H. Park, Y. C. Chung, J. H. Kim, B. I. Sang and Y. Um, Energy Fuels, 2008, 22(5), 3459–3464 CrossRef CAS
.
- S. Y. Lee, J. H. Park, S. H. Jang, L. K. Nielsen, J. Kim and K. S. Jung, Biotechnol. Bioeng., 2008, 101(2), 209–228 CrossRef CAS PubMed
.
-
J. Falbe, Carbon monoxide in organic synthesis, Springer Verlag, Berlin-Heidelberg-New York, 1970 Search PubMed
.
-
M. Bochman, F. A. Cotton, C. A. Murillo and G. Wilkinson, Advanced inorganic chemistry, John Wiley & Sons, Inc., USA, 1999 Search PubMed
.
- V. V. Zverlov, O. Berezina, G. A. Velikodvorskaya and W. H. Schwarz, Appl. Microbiol. Biotechnol., 2006, 71, 587–597 CrossRef CAS PubMed
.
- J. S. Chiao and Z. H. Sun, J. Mol. Microbiol. Biotechnol., 2007, 13, 12–14 CrossRef CAS PubMed
.
- Y. Ni and Z. Sun, Appl. Microbiol. Biotechnol., 2009, 83, 415–423 CrossRef CAS PubMed
.
- V. Garcia, J. Päkkilä, H. Ojamo, E. Muurinen and R. L. Keiski, Renewable Sustainable Energy Rev., 2011, 15, 964–980 CrossRef CAS PubMed
.
-
G. K. Donaldson, L. L. Huang, L. A. Maggio-Hall, V. Nagarajan, C. E. Nakamura and W. Suh, WO2007/041269 A2, 2007
.
-
M. Kirschner, Chemical Market Reporter, ABI/INFORM Global, 2006, p. 42.
- C. Jin, M. Yao, H. Liu, C. F. Lee and J. Ji, Renewable Sustainable Energy Rev., 2011, 15, 4080–4106 CrossRef CAS PubMed
.
-
n-Butanol Product Safety Assessment, Dow Chemical Company, April 2006 Search PubMed.
- US Energy Information Administration, Energy explained – 2009 statistics, http://www.eia.gov/energyexplained/, 2012.
-
EPA, Fuels and additives, http://www.epa.gov/oms/renewablefuels/420f09023.htm, 2012 Search PubMed
.
- A. J. Francis, C. J. Dodge, F. Lu, G. P. Halada and C. R. Clayton, Environ. Sci. Technol., 1994, 28, 636–639 CrossRef CAS PubMed
.
- D. T. Jones and D. R. Woods, Microbiol. Rev., 1986, 50, 484–524 CAS
.
-
P. Durre, in Handbook on Clostridia, ed. P. Durre, CRC Press, Boca Raton, 2005, pp. 659–699 Search PubMed
.
- W. Andersch, H. Bahl and G. Gottschalk, Eur. J. Appl. Microbiol. Biotechnol., 1983, 18, 327–332 CrossRef CAS
.
- M. G. N. Hartmanis and S. Gatenbeck, Appl. Microbiol. Biotechnol., 1984, 47, 1277–1283 CAS
.
- P. Durre, A. Kuhn, M. Gottwald and G. Gottschalk, Appl. Microbiol. Biotechnol., 1987, 26, 268–272 CrossRef
.
- R. C. Saxena, D. K. Adhikari and H. B. Goyal, Renewable Sustainable Energy Rev., 2009, 13, 167–178 CrossRef PubMed
.
- T. C. Ezeji, N. Qureshi and H. P. Blaschek, Process Biochem., 2007, 42, 34–39 CrossRef CAS PubMed
.
- F. Sabathe, A. Belaich and P. Soucaille, FEMS Microbiol. Lett., 2002, 217, 15–22 CrossRef CAS
.
- D. Antoni, V. V. Zverlov and W. H. Schwarz, Appl. Microbiol. Biotechnol., 2007, 77, 23–35 CrossRef CAS PubMed
.
- R. Koukiekolo, H. Y. Cho, A. Kosugi, M. Inui, H. Yukawa and R. H. Doi, Appl. Environ. Microbiol., 2005, 71(7), 3504–3511 CrossRef CAS PubMed
.
- S. Chauhan, Biomass Bioenergy, 2010, 34(9), 1300–1308 CrossRef PubMed
.
- N. Qureshi, T. C. Ezeji, J. Ebener, B. S. Dien, M. A. Cotta and H. P. Blaschek, Bioresour. Technol., 2008, 99, 5915–5922 CrossRef CAS PubMed
.
- N. Qureshi, B. C. Saha, R. E. Hector, S. R. Hughes and M. A. Cotta, Biomass Bioenergy, 2008, 32, 168–175 CrossRef CAS PubMed
.
- N. Qureshi, B. C. Saha and M. A. Cotta, Biomass Bioenergy, 2008, 32, 176–183 CrossRef CAS PubMed
.
- N. Qureshi, B. C. Saha, R. E. Hector and M. A. Cotta, Biomass Bioenergy, 2008, 32, 1353–1358 CrossRef CAS PubMed
.
- N. Qureshi, B. C. Saha, B. Dien, R. E. Hector and M. A. Cotta, Biomass Bioenergy, 2010, 34, 559–565 CrossRef CAS PubMed
.
- N. Qureshi, B. C. Saha, R. E. Hector, B. Dien, S. Hughes, S. Liu, L. Itena, M. J. Bowmana, G. Sarathc and M. A. Cotta, Biomass Bioenergy, 2010, 34(4), 566–571 CrossRef CAS PubMed
.
- N. Qureshi, B. C. Saha, B. Dien, R. E. Hector and M. A. Cotta, Biomass Bioenergy, 2010, 34(4), 559–565 CrossRef CAS PubMed
.
- T. C. Ezeji, N. Qureshi and H. P. Blaschek, Curr. Opin. Biotechnol., 2007, 18, 220–227 CrossRef CAS PubMed
.
- T. C. Ezeji, N. Qureshi and H. P. Blaschek, Biotechnol. Bioeng., 2007, 97(6), 1460–1469 CrossRef CAS PubMed
.
- S. Keis, C. F. Bennett, V. K. Ward and D. T. Jones, Int. J. Syst. Bacteriol., 1995, 45, 693–705 CrossRef CAS PubMed
.
- D. P. Wiesenborn, F. B. Rudolph and E. T. Papoutsakis, Appl. Environ. Microbiol., 1988, 54, 2717–2722 CAS
.
- Y. N. Zheng, L. Z. Li, M. Xian, Y. J. Ma, J. M. Yang, X. Xu and D. Z. He, J. Ind. Microbiol. Biotechnol., 2009, 36, 1127–1138 CrossRef CAS PubMed
.
- G. Jurgens, S. Survase, O. Berezina, E. Sklavounos, J. Linnekoski, A. Kurkijärvi, M. Väkevä, A. van Heiningen and T. Granström, Biotechnol. Lett., 2012, 34, 1415–1434 CrossRef CAS PubMed
.
- T. W. Jesse, T. C. Ezeji, N. Qureshi and H. P. Blaschek, J. Ind. Microbiol. Biotechnol., 2002, 29(3), 117–123 CrossRef CAS PubMed
.
- C. N. Hipolito, E. Crabbeb, C. M. Badilloa, O. C. Zarrabalc, M. A. M. Morad, G. P. Floresa, M. A. H. Cortazara and A. Ishizakie, J. Clean. Prod., 2008, 16(5), 632–638 CrossRef PubMed
.
- J. Lee, E. Seo, D. H. Kweon, K. Park and Y. S. Jin, J. Microbiol. Biotechnol., 2009, 19, 482–490 CrossRef CAS
.
- Z. Liu, Y. Ying, F. Li, C. Ma and P. Xu, J. Ind. Microbiol. Biotechnol., 2010, 37(5), 495–501 CrossRef CAS PubMed
.
- S. A. Survase, E. Sklavounos, G. Jurgens, A. van Heiningen and T. Granström, Bioresour. Technol., 2011, 102(23), 10996–11002 CrossRef CAS PubMed
.
- Y. Wang and H. P. Blaschek, Bioresour. Technol., 2011, 102(21), 9985–9990 CrossRef CAS PubMed
.
- Z. Sun and S. Liu, Biomass Bioenergy, 2012, 39, 39–47 CrossRef CAS PubMed
.
- J. Isar, H. Joshi and V. Rangaswamy, BioEnergy Res., 2013, 6, 991–999 CrossRef CAS
.
- N. Qureshi, M. J. Bowman, B. C. Saha, R. Hector, M. A. Berhow and M. A. Cotta, Food Bioprod. Process., 2012, 90, 533–540 CrossRef CAS PubMed
.
- Y. Sun, Y. Jin, X. Gao, X. Li, Y. Xiao and H. Zhao, Chin. J. Appl. Environ. Biol., 2010, 16(6), 845–850 CAS
.
- D. H. Cho, Y. J. Lee, Y. Um, B.-I. Sang and Y. H. Kim, Appl. Microbiol. Biotechnol., 2009, 83(6), 1035–1043 CrossRef CAS PubMed
.
- X. Mu, W. Sun, C. Liu and H. Wang, Biotechnol. Lett., 2011, 33(8), 1587–1591 CrossRef CAS PubMed
.
- L. Wang and H. Chen, Process Biochem., 2011, 46(2), 604–607 CrossRef CAS PubMed
.
- D. Cai, T. Zhang, J. Zheng, Z. Chang, Z. Wang, P.-Y. Qin and T.-W. Tan, Bioresour. Technol., 2013, 145, 97–102 CrossRef CAS PubMed
.
- T. Guo, Y. Tang, Y. L. Xi, A. Y. He, B. J. Sun, H. Wu, D. F. Liang, M. Jiang and P. K. Ouyang, J. Ind. Microbiol. Biotechnol., 2012, 99(3), 401–407 CrossRef PubMed
.
- T. Guo, A.-Y. He, T.-F. Du, D.-W. Zhu, D.-F. Liang, M. Jiang, P. Wei and P.-K. Ouyang, Bioresour. Technol., 2013, 135, 379–385 CrossRef CAS PubMed
.
- A. Tripathi, H. Sami, S. R. Jain, M. Viloria-Cols, N. Zhuravleva, G. Nilsson, H. Jungvid and A. Kumar, Enzyme Microb. Technol., 2010, 47, 44–51 CrossRef CAS PubMed
.
- E. N. Efremenko, N. A. Stepanov, A. B. Nikolskaya, O. V. Senko, O. V. Spiricheva and S. D. Varfolomeev, Catal. Ind., 2011, 3(1), 41–46 CrossRef
.
- S. Shamsudin, M. S. H. Kalil and W. M. W. Yusoff, Pak. J. Biol. Sci., 2006, 9(10), 1923–1928 CrossRef CAS
.
- S. A. Survase, A. van Heiningen and T. Granström, Appl. Microbiol. Biotechnol., 2012, 93(6), 2309–2316 CrossRef CAS PubMed
.
- F. Napoli, G. Olivieri, M. E. Russo, A. Marzocchella and P. Salatino, J. Ind. Microbiol. Biotechnol., 2010, 37, 603–608 CrossRef CAS PubMed
.
- S.-Y. Li, R. Srivastava, S. L. Suib, Y. Li and R. S. Parnas, Bioresour. Technol., 2011, 102(5), 4241–4250 CrossRef CAS PubMed
.
- K. Sonomoto, M. Oshiro, K. Hanada and Y. Tashiro, Appl. Microbiol. Biotechnol., 2010, 87(3), 1177–1185 CrossRef PubMed
.
- Y. Tashiro, K. Takeda, G. Kobayashi, K. Sonomoto, A. Ishizaki and S. Yoshino, J. Biosci. Bioeng., 2004, 98, 263–268 CAS
.
- T. C. Ezeji, N. Qureshi and H. P. Blaschek, Appl. Microbiol. Biotechnol., 2004, 63(6), 653–658 CrossRef CAS PubMed
.
- C. Lu, J. Zhao, S.-T. Yang and D. Wei, Bioresour. Technol., 2012, 104, 380–387 CrossRef CAS PubMed
.
- N. Qureshi, B. A. Annous, T. C. Ezeji, P. Karcher and I. S. Maddox, Microb. Cell Fact., 2005, 4, 24 CrossRef PubMed
.
- S. A. Survase, G. Jurgens, A. Van Heiningen and T. Granström, Appl. Microbiol. Biotechnol., 2011, 91(5), 1305–1313 CrossRef CAS PubMed
.
- H.-W. Yen and R.-J. Li, J. Chem. Technol. Biotechnol., 2011, 86(11), 1399–1404 CrossRef CAS
.
- Y. Tashiro, K. Takeda, G. Kobayashi and K. Sonomoto, J. Biotechnol., 2005, 120, 197–206 CrossRef CAS PubMed
.
- A. Malaviya, Y.-S. Jang and S. Y. Lee, Appl. Microbiol. Biotechnol., 2012, 93(4), 1485–1494 CrossRef CAS PubMed
.
- J. Zheng, Y. Tashiro, T. Yoshida, M. Gao, Q. Wang and K. Sonomoto, Bioresour. Technol., 2013, 129, 360–365 CrossRef CAS PubMed
.
- S. B. Bankar, S. A. Survase, R. S. Singhal and T. Granström, Bioresour. Technol., 2012, 106, 110–116 CrossRef CAS PubMed
.
- S. B. Bankar, S. A. Survase, H. Ojamo and T. Granström, Bioresour. Technol., 2013, 140, 269–276 CrossRef CAS PubMed
.
- M. Setlhaku, S. Heitmann, A. Górak and R. Wichmann, Bioresour. Technol., 2013, 136, 102–108 CrossRef CAS PubMed
.
- Y. Ni, Z. Xia, Y. Wang and Z. Sun, Bioresour. Technol., 2013, 129, 680–685 CrossRef CAS PubMed
.
- N. Qureshi and I. S. Maddox, Bioprocess Eng., 1988, 3, 69–72 CrossRef CAS
.
- F. W. Welsh, R. E. Williams and I. A. Veliky, Enzyme Microb. Technol., 1987, 9, 500–502 CrossRef CAS
.
- C. Forberg and L. Haggstrom, Enzyme Microb. Technol., 1985, 7(5), 230–234 Search PubMed
.
- C. Frick and K. Schugerl, Appl. Microbiol. Biotechnol., 1986, 25, 186–193 CrossRef CAS
.
- N. Qureshi, J. Schripsema, J. Lienhardt and H. P. Blaschek, World J. Microbiol. Biotechnol., 2000, 16, 377–382 CrossRef CAS
.
- N. Qureshi, P. Karcher, M. Cotta and H. P. Blaschek, Appl. Biochem. Biotechnol., 2004, 113–116, 713–721 CrossRef CAS
.
- J. Lienhardt, J. Schripsema, N. Qureshi and H. P. Blaschek, Appl. Biochem. Biotechnol., 2002, 98–100, 591–598 CrossRef CAS
.
- S. A. Survase, A. van Heiningen and T. Granström, J. Ind. Microbiol. Biotechnol., 2013, 40(2), 209–215 CrossRef CAS PubMed
.
- F. Raganati, G. Olivieri, A. Procentese, M. E. Russo, P. Salatino and A. Marzocchella, Bioresour. Technol., 2013, 138, 259–265 CrossRef CAS PubMed
.
- S. Liu and N. Qureshi, New Biotechnol., 2009, 26, 117–121 CrossRef CAS PubMed
.
- C. Lepage, F. Fayolle, M. Hermann and J. P. Vandecasteele, J. Gen. Microbiol., 1986, 133, 103–110 Search PubMed
.
- K. V. Schneck, J. A. Sands and B. S. Montenecourt, Appl. Environ. Microbiol., 1984, 47(1), 193–194 Search PubMed
.
- L. K. Bowles and W. Ellefson, Appl. Environ. Microbiol., 1985, 50(5), 1165–1170 CAS
.
- P. J. Evans and H. Y. Wang, Appl. Environ. Microbiol., 1988, 54, 1662–1667 CAS
.
- A. V. D. Westhuizen, D. T. Jones and D. R. Woods, Appl. Environ. Microbiol., 1982, 44(6), 1277–1281 Search PubMed
.
- O. V. Berezina, N. V. Zakharovaa, C. V. Yarotskya and V. V. Zverlov, Appl. Biochem. Microbiol., 2012, 48(7), 625–638 CrossRef CAS
.
- R. C. Woolley and J. G. Morris, J. Appl. Bacteriol., 1990, 69, 718–728 CrossRef CAS
.
- K. C. Schuster, R. Goodacre, J. R. Gapes and M. J. Young, J. Ind. Microbiol. Biotechnol., 2001, 27, 314–321 CrossRef CAS
.
- E. R. Kashket and Z. Y. Cao, Appl. Environ. Microbiol., 1993, 59, 4198–4202 CAS
.
- H. Liyanage, P. Holcroft, V. J. Evans, S. Keis, S. R. Wilkinson, E. R. Kashket and M. Young, J. Mol. Microbiol. Biotechnol., 2000, 2, 107–113 CAS
.
- D. T. Jones, M. Shirley, X. Wu and S. Keis, J. Mol. Microbiol. Biotechnol., 2000, 2, 21–26 CAS
.
- E. T. Papoutsakis, Curr. Opin. Biotechnol., 2008, 19, 420–429 CrossRef CAS PubMed
.
- F. Hillmann, C. Doring, O. Riebe, A. Ehrenreich, R. J. Fischer and H. Bahl, J. Bacteriol., 2009, 191(19), 6082–6093 CrossRef CAS PubMed
.
- R. W. O'Brien and J. G. Morris, J. Gen. Microbiol., 1971, 68, 307–318 CrossRef CAS PubMed
.
- W. J. Mitchell, J. E. Shaw and L. Andrews, Appl. Environ. Microbiol., 1991, 57, 534–539 Search PubMed
.
-
W. J. Mitchell and M. Tangney, in Handbook on Clostridia, ed. P. Duerre, CRC Press, Boca Raton, 2005, p.155 Search PubMed
.
- A. Ranjan and V. S. Moholkar, Fuel, 2011, 112, 567–571 CrossRef PubMed
.
-
A. Singh and P. Mishra, in Microbial Pentose Utilization: Current cations in Biotechnology, Elsevier Science, New York, 1995, vol. 33, p. 119 Search PubMed
.
- R. Gheshlaghi, J. M. Scharer, M. M. Young and C. P. Chou, Biotechnol. Adv., 2009, 27, 764–781 CrossRef CAS PubMed
.
- P. Durre, Biotechnol. J., 2007, 2(12), 1525–1534 CrossRef PubMed
.
- Y. Jiang, C. Xu, F. Dong, Y. Yang, W. Jiang and S. Yang, Metab. Eng., 2009, 11, 284–291 CrossRef CAS PubMed
.
- Y. Tashiro, H. Shinto, M. Hayashi, S. Baba, G. Kobayashi and K. Sonomoto, J. Biosci. Bioeng., 2007, 104(3), 238–240 CrossRef CAS PubMed
.
- R. Sillers, A. Chow, B. Tracy and E. T. Papoutsakis, Metab. Eng., 2008, 10, 321–332 CrossRef CAS PubMed
.
- H. Huang, H. Liu and Y.-R. Gan, Biotechnol. Adv., 2010, 28, 651–657 CrossRef CAS PubMed
.
- M. Inui, M. Suda, S. Kimura, K. Yasuda, H. Suzuki, H. Toda, S. Yamamoto, S. Okino, N. Suzuki and H. Yukawa, Appl. Microbiol. Biotechnol., 2008, 77, 1305–1316 CrossRef CAS PubMed
.
- D. R. Nielsen, E. Leonard, S. H. Yoon, H. C. Tseng, C. Yuan and K. L. Prather, Metab. Eng., 2009, 11, 262–273 CrossRef CAS PubMed
.
- P. Durre, Appl. Microbiol. Biotechnol., 1998, 49, 639–648 CrossRef CAS
.
- M. Kumar and K. Gayen, Appl. Energy, 2011, 88, 1999–2012 CrossRef CAS PubMed
.
- H. Bahl, W. Andersch and G. Gottschalk, Eur. J. Appl. Microbiol. Biotechnol., 1982, 15, 201–215 CrossRef CAS
.
- J. Ballongue, J. Amine, E. Masion, H. Petitdemange and R. Gay, FEMS Microbiol. Lett., 1986, 29(3), 273–277 CrossRef
.
- J. Ballongue, J. Amine, H. Petitdemange and R. Gay, FEMS Microbiol. Lett., 1986, 35(2–3), 295–301 CrossRef CAS
.
- B. K. Soni, K. Das and T. K. Ghosh, Biotechnol. Lett., 1982, 4, 19–22 CrossRef CAS
.
- E. Petitdemange, O. Fond, F. Caillet, H. Petitdemange and R. Gay, Biotechnol. Lett., 1983, 5, 119–124 CrossRef CAS
.
- E. Petitdemange, F. Caillet, J. Ciallo and C. Gaudin, Int. J. Syst. Bacteriol., 1984, 34, 155–159 CrossRef PubMed
.
- O. Fond, G. Matta-El-Amouri, J. M. Engasser and H. Petitdemange, Biotechnol. Bioeng. Symp., 1983, 13, 217–224 CAS
.
- S. L. Bergstrom and G. L. Foutch, Biotechnol. Bioeng. Symp., 1983, 13, 251–256 CAS
.
-
A. Spake, DuPont, http://www.usembassy.it/viewer/article.asp?article=/file2007_09/alia/a7091901.htm, 2007 Search PubMed.
- BP Biofuels News, http://www.bp.com/sectiongenericarticle.do?categoryId=9021783%26contentId=7041026, 2006.
- D. T. Jones and S. Keis, FEMS Microbiol. Rev., 1995, 17, 223–232 CrossRef CAS
.
- S. Kharkwal, I. A. Karimi, M. W. Chang and D. Y. Lee, Recent Pat. Biotechnol., 2009, 3, 202–210 CrossRef CAS
.
- I. S. Maddox, E. Steiner, S. Hirsch, S. Wessner, N. A. Gutierrez, J. R. Gapes and K. C. Schuster, J. Mol. Microbiol. Biotechnol., 2000, 2(1), 95–100 CAS
.
- D. H. Cho, S.-J. Shin and Y. H. Kim, Biotechnol. Bioprocess Eng., 2012, 17, 270–275 CrossRef CAS PubMed
.
- E. T. Papoutsakis, Biotechnol. Bioeng., 1984, 26, 174–187 CrossRef CAS PubMed
.
- S. Chauvatcharin, C. Siripatana, T. Seki, M. Takagi and T. Yoshida, Biotechnol. Bioeng., 1998, 58, 561–571 CrossRef CAS
.
-
G. F. Froment and K. B. Bischoff, Chemical reactor analysis and design, Wiley, New York, 1979 Search PubMed
.
-
O. Levenspiel, Chemical reaction engineering, Wiley, New York, 1972 Search PubMed
.
- L. Yerushalmi, B. Volesky and J. Votruba, Can. J. Chem. Eng., 1986, 64, 607–616 CrossRef CAS
.
- J. Votruba, B. Volesky and L. Yerushalmi, Biotechnol. Bioeng., 1986, 28, 247–255 CrossRef CAS PubMed
.
- A. Harder and J. A. Roels, Adv. Biochem. Eng., 1982, 21, 56–103 Search PubMed
.
- A. B. Jarzebski, G. Goma and P. Soucaille, Appl. Microbiol. Biotechnol., 1992, 37, 714–717 CrossRef CAS
.
- A. B. Jarzebski, G. Goma and P. Soucaille, Bioprocess Eng., 1992, 7, 357–361 CrossRef CAS
.
- J. W. Roos, J. K. McLaughlin and E. T. Papoutsakis, Biotechnol. Bioeng., 1985, 27, 681–694 CrossRef CAS PubMed
.
- M. H. W. Husemann and E. T. Papoutsakis, Biotechnol. Bioeng., 1988, 32, 843–852 CrossRef CAS PubMed
.
- B. K. Soni, K. Das, P. Soucaille and G. Goma, Biotechnol. Bioeng. Symp., 1986, 17, 591–603 CAS
.
- H. Honda, T. Mano, M. Taya, K. Shimizu, M. Matsubara and T. Kobayashi, Chem. Eng. Sci., 1987, 42, 493–498 CrossRef CAS
.
- H. Shinto, Y. Tashiro, M. Yamashita, G. Kobayashi, T. Sekiguchi, T. Hanai, Y. Kuriya, M. Okamoto and K. Sonomoto, J. Biotechnol., 2007, 131, 45–56 CrossRef CAS PubMed
.
- H. Shinto, Y. Tashiro, G. Kobayashi, T. Sekiguchi, T. Hanai, Y. Kuriya, M. Okamotod and K. Sonomoto, Process Biochem., 2008, 43, 1452–1461 CrossRef CAS PubMed
.
- Z. Shi and H. P. Blaschek, Appl. Environ. Microbiol., 2008, 74, 7709–7714 CrossRef CAS PubMed
.
- S. Atsumi and J. C. Liao, Curr. Opin. Biotechnol., 2008, 19, 414–419 CrossRef CAS PubMed
.
- S. Atsumi and J. C. Liao, Appl. Environ. Microbiol., 2008, 74, 7802–7808 CrossRef CAS PubMed
.
- S. Atsumi, A. F. Cann, M. R. Connor, C. R. Shen, K. M. Smith, M. P. Brynildsen, K. J. Y. Chou, T. Hanai and J. C. Liao, Metab. Eng., 2008, 10, 305–311 CrossRef CAS PubMed
.
- E. J. Steen, R. Chan, N. Prasad, S. Myers, C. J. Petzold, A. Redding, M. Ouellet and J. D. Keaslinget, Microb. Cell Fact., 2008, 7, 36–43 CrossRef PubMed
.
- M. Edward, Nature, 2008, 454, 841–845 CrossRef PubMed
.
- B. Meinecke, J. Bertram and G. Gottschalk, Arch. Microbiol., 1989, 152, 244–250 CrossRef CAS
.
- J.-M. Moulis, V. Davasse, J. Meyer and J. Gaillard, FEBS Lett., 1996, 380, 287–290 CrossRef CAS
.
- R. K. Thauer, K. Jungermann and K. Decker, Bacteriol. Rev., 1977, 41, 100–180 CAS
.
- E. Cornillot, R. V. Nair, E. T. Papoutsakis and P. Soucaille, J. Bacteriol., 1997, 179(17), 5442–5447 CAS
.
- C. R. Shen and J. C. Liao, Metab. Eng., 2008, 10, 312–320 CrossRef CAS PubMed
.
- D. P. Wiesenborn, F. B. Rudolph and E. T. Papoutsakis, Appl. Environ. Microbiol., 1989, 55, 317–322 CAS
.
- L. Sullivan, M. S. Cates and G. N. Bennett, Enzyme Microb. Technol., 2010, 46, 118–124 CrossRef CAS PubMed
.
- E. T. Papoutsakis and G. N. Bennett, Biotechnology, 1993, 25, 157–199 CAS
.
- R. W. Welch, F. B. Rudolph and E. T. Papoutsakis, Arch. Biochem. Biophys., 1989, 273, 309–318 CrossRef CAS
.
- L. D. Mermelstein, E. T. Papoutsakis, D. J. Petersent and G. N. Bennett, Biotechnol. Bioeng., 1993, 42, 1053–1060 CrossRef CAS PubMed
.
- L. M. Harris, R. P. Desai and N. E. Welker, Biotechnol. Bioeng., 2000, 67, 1–11 CrossRef CAS
.
- S. B. Tummala, N. E. Welker and E. T. Papoutsakis, J. Bacteriol., 2003, 185, 1923–1934 CrossRef CAS PubMed
.
- A. R. Moreira, D. C. Ulmer and J. C. Linden, Biotechnol. Bioeng. Symp., 1981, 11, 567–579 CAS
.
- H. Lyanage, M. Young and E. R. Kashket, J. Mol. Microbiol. Biotechnol., 2000, 2, 87–93 Search PubMed
.
- K. V. Alsaker, T. R. Spitzer and E. T. Papoutsakis, J. Bacteriol., 2004, 186, 1959–1971 CrossRef CAS PubMed
.
- C. A. Tomas, J. Beamish and E. T. Papoutsakis, J. Bacteriol., 2004, 186, 2006–2018 CrossRef CAS
.
- J. R. Borden and E. T. Papoutsakis, Appl. Environ. Microbiol., 2007, 73, 3061–3068 CrossRef CAS PubMed
.
- C. A. Tomas, N. E. Welker and E. T. Papoutsakis, Appl. Environ. Microbiol., 2003, 69, 4951–4965 CrossRef CAS
.
- L. M. Harris, N. E. Welker and E. T. Papoutsakis, J. Bacteriol., 2002, 184, 3586–3597 CrossRef CAS
.
- M. C. Scotcher, F. B. Rudolph and G. N. Bennett, Appl. Environ. Microbiol., 2005, 71, 1987–1995 CrossRef CAS PubMed
.
- K. V. Alsaker and E. T. Papoutsakis, J. Bacteriol., 2005, 187, 7103–7118 CrossRef CAS PubMed
.
- S. W. Jones, C. J. Paredes, B. Tracy, N. Cheng, R. Sillers, R. S. Senger and E. T. Papoutsakis, GenomeBiology, 2008, 9, R114 CrossRef PubMed
.
- C. Grimmler, H. Janssen, D. Kraubetae, R. J. Fischer, H. Bahl, P. Dürre and W. Liebl, J. Mol. Microbiol. Biotechnol., 2011, 20, 1–15 CrossRef CAS PubMed
.
- J. Y. Lee, Y. S. Jang, J. Lee, E. T. Papoutsakis and S. Y. Lee, Biotechnol. J., 2009, 4, 1432–1440 CrossRef CAS PubMed
.
- R. V. Nair and E. T. Papoutsakis, J. Bacteriol., 1994, 176, 5843–5846 CAS
.
- S. W. Clark, G. N. Bennett and F. B. Rudolph, Appl. Environ. Microbiol., 1989, 55, 970–976 CAS
.
- O. V. Berezina, N. V. Zakharova, A. Brandt, S. V. Yarotsky, W. H. Schwarz and V. V. Zverlov, Appl. Microbiol. Biotechnol., 2010, 87, 635–646 CrossRef CAS PubMed
.
- E. P. Knoshaug and M. Zhang, Appl. Biochem. Biotechnol., 2009, 153, 13–20 CrossRef CAS PubMed
.
- Y.-S. Jang, J. Lee, A. Malaviya, D. Y. Seung, J. H. Cho and S. Y. Lee, Biotechnol. J., 2012, 7, 186–198 CrossRef CAS PubMed
.
-
G. Jurgens, T. B. Granström and A. van Heiningen, Cloning and expression of primary-secondary alcohol dehydrogenase gene from Clostridium beijerinckii as a part of the project of producing biofuels from forest biomass, Poster at Clostridium 11 conference, San Diego, 2010 Search PubMed
.
- J. T. Heap, M. Ehsaan, C. M. Cooksley, Y.-K. Ng, S. T. Cartman, K. Winzer and N. P. Minton, Nucleic Acids Res., 2012, 40(8), e59 CrossRef CAS PubMed
.
- J. Lee, Y. S. Jang, S. J. Choi, J. A. Im, H. Song, J. H. Cho, Y. Seung do, E. T. Papoutsakis, G. N. Bennett and S. Y. Lee, Appl. Environ. Microbiol., 2012, 78(5), 1416–1423 CrossRef CAS PubMed
.
- E. Sklavounos, M. Iakovlev, M. Yamamoto, L. Teräsvuori, G. Jurgens, T. Granström and A. van Heiningen, Holzforschung, 2011, 65(4), 551–558 CrossRef CAS
.
- Y. L. Lin and H. P. Blaschek, Appl. Environ. Microbiol., 1983, 45(3), 966–973 CAS
.
- Q. Syed, M. Nadeem and R. Nelofer, Turk. J. Biochem., 2008, 33(1), 25–30 CAS
.
- B. A. Annous and H. P. Blaschek, Appl. Environ. Microbiol., 1991, 57(9), 2544–2548 CAS
.
- J. Formanek, R. Mackie and H. P. Blaschek, Appl. Environ. Microbiol., 1997, 63(6), 2306–2310 CAS
.
- M. R. Connor, A. F. Cann and J. C. Lio, Appl. Microbiol. Biotechnol., 2010, 86, 1155–1164 CrossRef CAS PubMed
.
- B. B. Bond-Watts, R. J. Bellerose and M. C. Y. Chang, Nat. Chem. Biol., 2011, 7, 222–227 CrossRef CAS PubMed
.
- J. Nielsen, Nat. Chem. Biol., 2011, 7, 195–196 CrossRef CAS PubMed
.
- B. Jong, V. Siewers and J. Nielsen, Curr. Opin. Biotechnol., 2012, 23, 624–630 CrossRef PubMed
.
- A. Krivoruchko, V. Siewers and J. Nielsen, Biotechnol. J., 2011, 6, 262–276 CrossRef CAS PubMed
.
- C. R. Fischer, D. Klein-Marcuschamer and G. Stephanopoulos, Metab. Eng., 2008, 10, 295–304 CrossRef CAS PubMed
.
- F. Matsuda, C. Furusawa, T. Kondo, J. Ishii, H. Shimizu and A. Kondo, Microb. Cell Fact., 2011, 10, 70 CrossRef CAS PubMed
.
-
U. Gunawardena, P. Meinhold, M. Peters, J. Urano and R. Feldman, US 2010/0062505 A1, 2010
.
-
C. Gerday and N. Glansdorff, Physiology and Biochemistry of Extremophiles, ASM Press, Washington, DC, 2007 Search PubMed
.
- B. S. Dien, M. A. Cotta and T. W. Jeffries, Appl. Microbiol. Biotechnol., 2003, 63, 258–266 CrossRef CAS PubMed
.
- J. Zaldivar, J. Nielsen and L. Olsson, Appl. Microbiol. Biotechnol., 2001, 56, 17–34 CrossRef CAS
.
- D. Barnard, A. Casanueva, M. Tuffin and D. Cowan, Environ. Technol., 2010, 31(8–9), 871–888 CrossRef CAS PubMed
.
- Y. Sardessai and S. Bhosle, Res. Microbiol., 2002, 153, 263–268 CrossRef CAS
.
- A. Segura, E. Duque, A. Rojas, P. Godoy, A. Delgado, A. Hurtado, J. Cronan and J. L. Ramos, Environ. Microbiol., 2004, 6, 416–423 CrossRef CAS
.
- J. L. Ramos, E. Duque, M. T. Gallegos, P. Godoy, M. I. Ramos-Gonzales, A. Rojas, W. Teran and A. Segura, Annu. Rev. Microbiol., 2002, 56, 743–768 CrossRef CAS PubMed
.
- J. Rühl, A. Schmid and L. M. Blank, Appl. Environ. Microbiol., 2009, 75, 4653–4656 CrossRef PubMed
.
- C. C. de Carvalho, A. A. da Cruz, N. M. Pons, H. M. Pinheiro, J. M. Cabral, M. M. da Fonseca, B. S. Ferreira and P. Fernandes, Microsc. Res. Tech., 2004, 15, 215–222 CrossRef PubMed
.
- S. Atsumi, T. Hanai and J. C. Liao, Nature, 2008, 451, 86–99 CrossRef CAS PubMed
.
- A. Garcia, E. L. Lannotti and J. L. Fischer, Biotechnol. Bioeng., 1986, 28, 785–791 CrossRef CAS PubMed
.
- S. Nakayama, T. Morita, H. Negishi, T. Ikegami, K. Sakaki and D. Kitamoto, FEMS Yeast Res., 2008, 8(5), 706–714 CrossRef CAS PubMed
.
- N. Qureshi and H. P. Blaschek, Renewable Energy, 2001, 22, 557–564 CrossRef CAS
.
- N. Qureshi and H. P. Blaschek, J. Ind. Microbiol. Biotechnol., 2001, 27, 292–297 CrossRef CAS
.
- N. B. Milestone and D. M. Bibby, J. Chem. Technol. Biotechnol., 1981, 31, 732–736 CrossRef CAS
.
- W. J. Groot and K. C. A. M. Luyben, Appl. Microbiol. Biotechnol., 1986, 25, 29–31 CrossRef CAS
.
- M. Larson and B. Mattiasson, Chem. Ind., 1984, 12, 428–431 Search PubMed
.
- L. Nielsen, M. Larsson, O. Holst and B. Mattiasson, Appl. Microbiol. Biotechnol., 1988, 28, 335–339 CrossRef CAS
.
- S. R. Roffler, H. W. Blanch and C. R. Wilke, Bioprocess Eng., 1987, 2, 1–12 CrossRef CAS
.
- G. Eckert and K. Schugerl, Appl. Microbiol. Biotechnol., 1987, 27, 221–228 CrossRef CAS
.
- P. J. Evans and H. Y. Wang, Appl. Microbiol. Biotechnol., 1990, 32, 393–397 CrossRef CAS
.
- B. H. Davidson and J. E. Thompson, Appl. Biochem. Biotechnol., 1993, 39–40, 415–426 CrossRef
.
- N. Qureshi and I. S. Maddox, Food Bioprod. Process., 2005, 83, 43–52 CrossRef CAS
.
- W. J. Groot, R. G. J. M. van der Lans and K. C. A. M. Luyben, Process Biochem., 1992, 27, 61–75 CrossRef CAS
.
- M. Matsumura and H. Kataoka, Biotechnol. Bioeng., 1987, 30, 887–895 CrossRef CAS PubMed
.
-
T. C. Ezeji, N. Qureshi and H. P. Blaschek, in Handbook on Clostridia, ed. P. Durre, CRC Press, Taylor & Francis Group, Boca Raton, Florida, 2005, pp. 797–812 Search PubMed
.
-
T. C. Ezeji, N. Qureshi and H. P. Blaschek, US Provisional Patent No. 60/504,280, 2005
.
-
T. C. Ezeji, N. Qureshi, P. Karcher and H. P. Blaschek, in Alcoholic Fuels: Fuels for Today and Tomorrow, ed. S. D. Minteer, Taylor & Francis, New York, NY, 2006, pp. 99–122 Search PubMed
.
- A. P. Mariano and R. M. Filho, BioEnergy Res., 2012, 5, 504–514 CrossRef CAS
.
- N. Qureshi, M. M. Meagher, J. Huang and R. W. Hutkins, J. Membr. Sci., 2001, 187, 93–102 CrossRef CAS
.
- N. Qureshi, S. Hughes, I. S. Maddox and M. A. Cotta, Bioprocess Biosyst. Eng., 2005, 27, 215–222 CrossRef CAS PubMed
.
- A. Demirdas, Appl. Energy, 2009, 86, 108–117 CrossRef PubMed
.
- N. Qureshi and I. S. Maddox, Appl. Biochem. Biotechnol., 1992, 34–35, 441–448 CrossRef CAS
.
- T. G. Lenz and A. R. Moreira, Ind. Eng. Chem. Prod. Res. Dev., 1980, 19, 478–483 CrossRef CAS
.
- N. Qureshi and H. P. Blaschek, Food Bioprod. Process., 2000, 78, 139–144 CrossRef
.
- J. A. Marlatt and R. Datta, Biotechnol. Prog., 1986, 2(1), 23–28 CrossRef CAS PubMed
.
- J. R. Gapes, J. Microbiol. Biotechnol., 2000, 2(1), 27–32 CAS
.
-
FO Lichts ethanol production costs: a worldwide survey, Agra Informa, Tunbridge Wells, Kent, 2007 Search PubMed.
- D. R. Woods, Trends Biotechnol., 1995, 13, 259–264 CrossRef CAS
.
-
World Petrochemicals Report, Oxo Alcohols, SRI Consulting, Englewood, CA, USA, 2009, pp. 17 and 181 Search PubMed.
- ICIS Pricing Chemical Price Report on oxo-alcohols.
- M. Mascal, Biofuels, Bioprod. Biorefin., 2012, 6(4), 483–493 CrossRef CAS
.
- E. I. Khamaiseh, M. S. Kalil, O. Dada, I. El-Shawabkeh and W. M. W. Yusoff, J. Appl. Sci., 2012, 12(11), 1160–1165 CrossRef CAS
.
- N. Qureshi, B. C. Saha and M. A. Cotta, Bioprocess Biosyst. Eng., 2007, 30, 419–427 CrossRef CAS PubMed
.
- W. L. Zhang, Z. Y. Liu, Z. Liu and F. L. Li, Lett. Appl. Microbiol., 2012, 55(3), 240–246 CrossRef CAS PubMed
.
- S. Linggang, L. Y. Phang, H. Wasoh and S. Abd-Aziz, BioEnergy Res., 2013, 6(1), 321–328 CrossRef CAS
.
- T. C. Ezeji and H. P. Blaschek, Bioresour. Technol., 2008, 99, 5232–5242 CrossRef CAS PubMed
.
- W. C. Huang, D. E. Ramey and S. T. Yang, Appl. Biochem. Biotechnol., 2004, 113–116, 887–898 CrossRef CAS
.
- Y. Zhang, Y. Ma, F. Yang and C. Zhang, J. Ind. Microbiol. Biotechnol., 2009, 36, 1117–1121 CrossRef CAS PubMed
.
- H. R. Badr, R. Toledo and M. K. Hamdy, Biomass Bioenergy, 2001, 20, 119–132 CrossRef CAS
.
- E. M. Green, Curr. Opin. Biotechnol., 2011, 22(3), 337–343 CrossRef CAS PubMed
.
|
This journal is © The Royal Society of Chemistry 2013 |