DOI:
10.1039/C3RA40497H
(Review Article)
RSC Adv., 2013,
3, 12003-12020
Superhydrophobic surfaces for the reduction of bacterial adhesion
Received
29th January 2013
, Accepted 30th March 2013
First published on 4th April 2013
Abstract
As an important research area, the development of antibacterial materials has attracted extensive interest from researchers. Typical antibacterial materials involve the use of biocides and antibacterial metallic ions, such as Ag+, as well as killing by highly reactive species, such as hydroxyl radical, hydrogen peroxide and superoxide produced by the photocatalysis of TiO2. However, the intensive usage of biocides has a growing concern in the increase of bacterial resistance and cross-resistance to antibiotics and antibacterial Ag+ depending on its dissolution property may have potential implications on human health and environment. Currently TiO2 is mainly activated with UVA light and research on visible light photocatalysis is still under development. Recently, a new scheme using superhydrophobicity has raised more attention and interests especially for its ability in reducing bacterial adhesion. This paper provides a detailed review on the basics, recent developments, existing challenges and future perspectives of superhydrophobic surfaces especially in reducing bacterial adhesion.
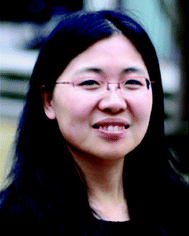 Xiaoxue Zhang | Dr Xiaoxue Zhang is a Postdoctoral Research Fellow in Department of Materials Science at Tampere University of Technology (TUT), Finland. She is now also a visiting scholar at the national Centre for Advanced Tribology at the University of Southampton, UK. She received her BSc from Beijing University of Technology, China, MSc and PhD (with distinction) in materials science from TUT. She has published 25 full papers including 20 peer-reviewed journal articles. Her main research areas are superhydrophobic multifunctional surfaces, textured surfaces with low friction, processing of materials for energy and environmental applications by sol–gel, hydrothermal, supercritical fluids methods, and materials characterization. |
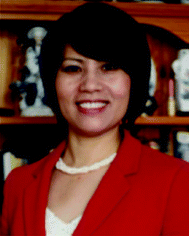 Ling Wang | Dr Ling Wang is a lecturer at the national Centre for Advanced Tribology (nCATS) at the University of Southampton (UoS). She received her BSc and MSc in Chemistry from Nankai University China and her PhD in system engineering from Nottingham Trent University UK before she joined UoS in 2001. Her research focuses on surface engineering, tribology test design, sensing and condition monitoring for bearings, industrial lubricants and rotating machinery using vibration, acoustic emission and electrostatic sensing techniques. |
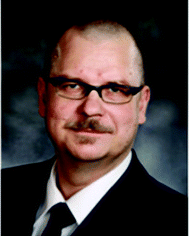 Erkki Levänen | Dr Erkki Levänen is Professor of Ceramic Materials at the Department of Materials Science at Tampere University of Technology since 2012. Professor Levänen's research interests are focused on functional ceramics especially for energy and environmental applications. His work ranges from material synthesis to novel processing techniques and advanced characterization methods as well as application oriented research. Nanoparticle and thin film synthesis include sol–gel, supercritical carbon dioxide assisted synthesis and pyrolysis methods aiming to multifunctional materials with self-cleaning, antibacterial, photoactive, bioactive and catalytic properties. Professor Levänen is currently author of 150 publications from which 45 are peer-reviewed. |
1 Introduction
The adhesion and proliferation of bacteria on abiotic surfaces and the subsequent biofilm formation pose challenges in both healthcare and industrial applications. The design of antibacterial materials has been a long-standing effort and a number of strategies have been developed to limit bacterial colonization on material surfaces. The first approach, as the oldest method for antibacterial surface design, focuses on leaching biocides (for example cytotoxic compounds from a surface) and inducing the death of both the adhered bacteria and nearby bacteria.1 However, while the biocides are widely used in everyday life to limit potential sources of infection, there is a growing concern that an increasing use of biocides can result in higher bacterial resistance and the cross-resistance to clinically important antibiotics.2 It has thus been suggested that biocides should be much more prudently used.3–5 Antibacterial metals, such as Ag,6 Cu7 and Mo,8 with Ag being the oldest and most popular one, are also used as a type of antibacterial agent to induce the death of adhered bacteria by their metallic ions. The antibacterial effect of Ag is mainly based on Ag+ ions and therefore dependent on the dissolution rate of Ag.6 When Ag is dissolved completely from the surface, its antibacterial effect will be gone. Besides, there are also growing concerns on its bacterial resistance and its potential implications for human health and the environment.9,10
More recently, strategies which do not involve the use of biocides and antibacterial metallic ions have also been developed. One approach uses photocatalytic materials such as TiO2 to produce highly reactive species such as hydroxyl radical, hydrogen peroxide and superoxide to kill bacteria.11 This method has been successful in killing Escherichia coli cells and some other types of bacteria.12,13 However, TiO2 is mainly active with UVA light and research is being carried out in activating TiO2 using visible light.14,15
Developing antibacterial materials based on superhydrophobicity is a new strategy that has been recently developed. Traditionally, only the materials that can induce the death of bacteria have been referred to as antibacterial materials. It is more and more acceptable to include superhydrophobic surfaces in this category even though they reduce bacterial adhesion rather than killing them directly.16–18 Superhydrophobicity can reduce the adhesion force between bacteria and a solid surface to enable the easy removal of bacteria before a thick biofilm is formed on the surface.19 This paper provides a detailed review on the basics, recent developments, existing challenges and future perspectives of superhydrophobic surfaces especially in reducing bacterial adhesion for antibacterial applications. In the following sections, the basics of superhydrophobic surfaces and wetting are firstly explained, followed by a summary of typical materials properties that affect bacterial adhesion on abiotic surface to provide an overview on the material-bacteria interaction. The fourth section highlights research on superhydrophobic surfaces that have reduced the adhesion of a variety of bacteria. Finally the challenges and future trends in applying superhydrophobic surfaces for the reduction of bacterial adhesion are discussed.
2 Superhydrophobic surfaces
The superhydrophobic surface is inspired by the Lotus leaf in nature, where a water contact angle of over 150° is observed.20 It was suggested in 1997 that the Lotus leaf has a microscale surface topography that comprises convex microstructures (papillose epidermal cells) immersed in a dense layer of epicuticular waxes.21 In 2002, it was further revealed that the Lotus leaf has a hierarchical micro/nanostructure.22 Since then it has been commonly accepted that the superhydrophobic characteristic of the Lotus leaf is due to its hierarchical micro/nanostructures and the hydrophobic wax on top of the surface. Generally, water forms droplets on a Lotus leaf and rolls off easily when it is tilted. Research on superhydrophobic surfaces has attracted a significant amount of interest and its rapid progress is clearly shown in the fast growth of scientific publications. Fig. 1 shows the results of a search on the ISI Web of Knowledge using “superhydrophobic”, “super hydrophobic”, “superhydrophobicity” or “super hydrophobicity” in the titles of the publications.
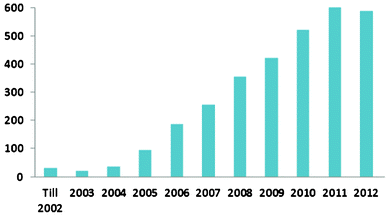 |
| Fig. 1 The number of scientific publications with “superhydrophobic”, “super hydrophobic”, “superhydrophobicity” or “super hydrophobicity” in their titles from the ISI Web of Knowledge. | |
2.1 Wetting phenomena
2.1.1 Wetting models.
Wetting reflects how a liquid behaves on a solid surface. Young's equation is typically used to describe wetting on an ideal smooth surface. By assuming the solid surface is smooth, rigid, chemically homogeneous, insoluble and non-reactive, the contact angle (θ, the angle between γsl and γlv, in Fig. 2a) can be defined by Young's equation23,24 as follows: | 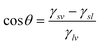 | (1) |
where θ is Young's contact angle, γ is the surface tension defined as the force per unit length of the interface and s, l and v represent the solid, liquid and vapour respectively. Therefore, γsv is the surface tension between the solid phase and the vapour phase, γsl between the solid phase and the liquid phase, and γlv between the liquid phase and the vapour phase (see Fig. 2a). γ is also often referred to as the surface energy or surface free energy in literature, which is the amount of energy applied to break chemical bonds. Molecules that do not form chemical bonds on the surface tend to have a higher energy, i.e. surface free energy, than those that form chemical bonds.25 The surface free energy is measured in unit of Joules per square meter (J m−2), which is equivalent to a surface tension measured in Newtons per meter (N m−1). Though thermodynamically the surface tension and surface free energy are different, they are numerically equivalent and often referred to as the same thing in literature.20 However, in practice, in dealing with liquids, the surface tension is usually used while the surface free energy is a more general term for solids.
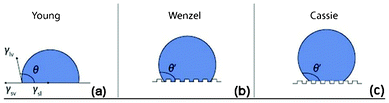 |
| Fig. 2 Illustration of a liquid droplet on an ideal smooth surface in (a), on a rough surface in a Wenzel regime in (b) and on a rough surface in a Cassie–Baxter regime in (c). Reprinted by kind permission from Macmillan Publishers Ltd: NPG Asia Materials, A. Nakajima, Design of hydrophobic surfaces for liquid droplet control, 2011, 3, 49–56, Copyright (2011). | |
In real situations, solid surfaces are usually rough and chemically heterogeneous. Wetting on a rough surface often takes place in two different regimes, as shown in Fig. 2b and 2c. In Fig. 2b, the liquid has completely penetrated into the roughness grooves and it is called homogeneous wetting. In Fig. 2c, there is air entrapped in the roughness grooves underneath the liquid and it is called heterogeneous wetting.16 On a superhydrophobic surface, water usually has heterogeneous wetting where air is entrapped between the water droplets and the surface.
Homogeneous wetting can be described by Wenzel equation26 below,
where
θA is the apparent contact angle,
r is the roughness parameter (or roughness ratio) defined as the ratio of the actual contacting area of a rough surface to the geometric projected area, and
θY is Young’s contact angle on a flat surface. The apparent contact angle was introduced for wetting on rough surfaces because the actual contact angle is often inaccessible. The apparent contact angle is the angle between the tangent to the liquid-air interface and the line that represents the normal solid–liquid interface.
27 The difference between the two contact angles could be large. The Wenzel equation also states that both hydrophilicity and hydrophobicity are enhanced by roughness, that is a rough hydrophilic surface (
θY < 90°) becomes more hydrophilic, and a rough hydrophobic surface (
θY > 90°) more hydrophobic than a smooth surface with the same chemical composition.
28
On the other hand, heterogeneous wetting can be described by the Cassie–Baxter equation29 below,
where
θA is the apparent contact angle,
f1 is the fraction of solid material in contact with liquid,
θ1 the contact angle of the pure solid material and
f2 the fraction of air in contact with the liquid (
f1 +
f2 = 1).
It is also suggested that heterogeneous regime on a superhydrophobic surface is thermodynamically metastable, while homogenous regime is stable.30 The metastability or the regime transition can be demonstrated in several ways. For instance, by applying a small pressure on the metastable Cassie–Baxter droplet, the droplet can slip to the stable Wenzel regime. Similarly, a Cassie–Baxter droplet can recede into a Wenzel droplet by allowing a small amount of the liquid to evaporate. The state of the droplet also depends on the amount of liquid and the means of depositing the liquid on the surface. For instance, when the liquid is delivered in the form of a mist, it wets the surface instantly as a Wenzel droplet.16
2.1.2 Contact angle, contact angle hysteresis, roll-off angle and sliding angle.
When the contact angle (θ) is 0°, the droplet spreads completely on the solid surface and this is referred to as complete wetting. On the contrary, when θ is 180° the droplet stands on the surface and it is named as non-wetting. The above two cases are the two extremes. When water is used as the liquid, the surface is hydrophilic when the contact angle is less than 90°. Superhydrophilic surfaces are those with contact angles for water less than 5°.31 On the other hand, a surface is hydrophobic when the contact angle for water is above 90° and superhydrophobic when it is more than 150°.
Contact angle hysteresis means that two different contact angles exist along the contact line of a droplet, as shown in Fig. 3, where the advancing angle θa at the front of the drop is larger than the receding angle θr at the rear of the drop.32 The contact angle hysteresis is therefore defined as the difference between the advancing and receding angles:
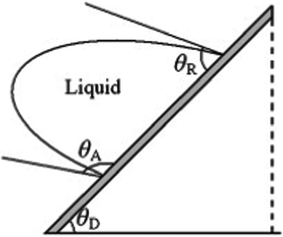 |
| Fig. 3 Illustration of a droplet moving along an inclined surface with advancing angle θa and receding angle θr. Reprinted from Advances in Colloid and Interface Science, 2011, 169, Y. Y. Yan, N. Gao and W. Barthlott, Mimicking natural superhydrophobic surfaces and grasping the wetting process: A review on recent progress in preparing superhydrophobic surfaces, pages 80–105, Copyright (2011), with kind permission from Elsevier. | |
Contact angle hysteresis is an important parameter in understanding drop motion on a surface. In practice, hysteresis is caused by surface roughness, contamination, chemical heterogeneity, drop size, molecular orientation and deformation, and liquid molecular transport.
In addition to the hysteresis, the roll-off angle or tilt angle is also defined as the angle at which the droplet starts to roll off from an inclined surface.30 Surfaces with low roll-off angle or tilt angle tend to have low contact angle hysteresis.33 In most cases, water has very low roll-off angles of a few degrees on superhydrophobic surfaces. However, sticky superhydrophobic surfaces with high contact angle hysteresis have also been reported.34 The critical angle at which the droplet with a certain weight begins to slide down the inclined surface is defined as the sliding angle.35 Typically rolling only occurs on a superhydrophobic surface, while sliding happens on other surfaces if the droplet could move.
2.1.3 Surface free energy.
Various approaches have been used to determine the surface free energy of solid materials. In the Owens and Wendt geometric mean approach, the surface free energy is divided into two components: dispersive and polar.36 The resulting equation when combined with Young's equation is: | γL (1 + cosθ) = 2 [(γLpγsp)1/2 + (γLdγsd)1/2] | (5) |
where θ is the contact angle, γL is the liquid surface tension and γs is the solid surface tension or surface free energy. The additions of d and p in the superscripts refer to the dispersive and polar components of each. The total surface free energy is merely the sum of its two components. To obtain the γsd and γsp of a solid, the contact angles of at least two liquids with known surface tension components (γL, γLp, γLd) on the solid must be determined.37 On the other hand, if the liquid has only the polar component in its surface tension, and the solid only has the dispersive component in its surface tension, wetting will not happen, and vice versa.
The other well accepted approach is the acid–base theory (LW/AB theory) proposed by van Oss et al.38 In their approach, the surface free energy is decomposed into γLW and γAB:
| γ = γLW + γAB, with γAB = 2(γ+γ−)1/2 | (6) |
LW stands for Lifshitz–van der Waals interactions, which include the London dispersion, Keesom dipole–dipole and Debye induction. AB represents Lewis acid–base interactions.
γ+ and
γ− are the Lewis acid (electron-acceptor) and Lewis base (electron-donor) parameters of the surface free energy, respectively. The larger
γ+ is, the stronger ability it has to accept electrons. On the other hand, the larger
γ− is, the stronger ability it has to provide electrons. In order to determine the three unknowns (
γLW,
γ+,
γ−), three test liquids with known surface energy parameters are required.
Surface energy is an intrinsic property of materials. Low surface energy molecules include primarily methylated and fluorinated carbons, with surface energy decreasing in the following manner: –CH2– > –CH3 > –CF2– > –CF2H > –CF3. The lowest surface energy of any readily available solid is 6.7 mN m−1 based on the hexagonal closed alignment of –CF3 groups on the surface, which gives a water contact angle of 119° on a smooth surface.39 Therefore, in order to achieve a contact angle for water of over 119°, a rougher surface is required, according to the Wenzel equation.
2.1.4 Free energy of interaction.
Instead of using the contact angle, another way of characterizing surface hydrophobicity and hydrophilicity is to calculate the free energy of interaction between a surface (i) and water (w) (ΔGiwi) using the surface energy components. The equation is as follows:40 |  | (7) |
By definition, hydrophobic surfaces are those with ΔGiwi < 0, whereas hydrophilic surfaces are those with ΔGiwi > 0. Superhydrophobic surfaces are those with ΔGiwi < −84.
2.2 Lotus effect: self-cleaning
The Lotus effect was introduced in 1997 to explain the self-cleaning property of the Lotus leaf.41 For centuries the Lotus has been the symbol of cleanliness in Asian culture. Due to the low surface energy of the waxes and the surface topography shown in Fig. 4a, the Lotus leaf has a very high water contact angle of 170° and a very low roll-off angle of a few degrees. On such a surface, air is entrapped in most of the surface area, hence significantly reducing the contact area between water and the leaf surface as well as that between the dirt particles and the leaf surface. This considerably reduces the adhesion force of water as well as the dirt particles to the leaf surface. Therefore the adhesion of dirt particles to the leaf surface is much weaker than that to the water droplet. When the water droplet rolls off from the leaf surface, dirt particles are taken away, as illustrated in Fig. 4b and Fig. 5a. This phenomenon has been referred to as the Lotus effect. On the contrary, on a titled smooth surface the water droplet tends to slide off the surface. It passes through the dirt particles leaving the particles on the surface (illustrated in Fig. 5b).
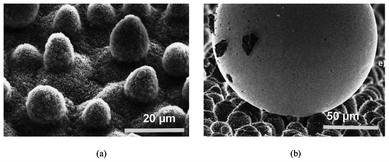 |
| Fig. 4 A scanning electron microscopy image of a Lotus leaf (Nelumbo nucifera) in (a) and an illustration of the reduced contact area of a mercury droplet on the leaf surface of a Colocasia esculenta and the contaminating particles adhering to the mercury droplet in (b). Reprinted from W. Barthlott and C. Neinhuis, Purity of the sacred lotus, or escape from contamination in biological surfaces, Planta 1997, 202, 1–8, Copyright (1997), with kind permission from Springer Science and Business Media. | |
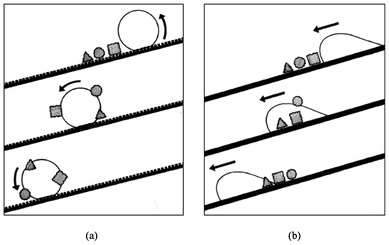 |
| Fig. 5 Illustration of a water droplet rolling off a rough surface in (a) and sliding on a flat surface in (b). Reprinted from W. Barthlott and C. Neinhuis, Purity of the sacred lotus, or escape from contamination in biological surfaces, Planta 1997, 202, 1–8, Copyright (1997), with kind permission from Springer Science and Business Media. | |
To be more precise, a superhydrophobic surface is easy-to-clean rather than self-clean. Zhang et al.42 tested a superhydrophobic surface in a paper machine and revealed that the superhydrophobic surface was not self-cleaning (being clean by itself) but covered by contaminants from the paper machine environment. However, the contaminants could be easily cleaned by washing with pressure water due to the low adhesion force of the contaminants to the surface.
2.3 Other functionalities
In addition to the easy-to-clean property, superhydrophobic surfaces have been found to be able to reduce bacterial adhesion and have an easy removal capability of the bacterial cells slowing down the thick biofilm formation processes. Superhydrophobic surfaces are also believed to have other functionalities such as water repellence, broadband anti-reflection,43,44 anti-icing,45–47 drag-reduction in fluid flow,48,49 non-adhesive property50 and wetting control.51,52
2.4 General processing schemes
A number of processes for generating superhydrophobic surfaces have been extensively reported in the last two decades. It has been commonly accepted that low surface energy and surface roughness are the two dominant factors in the processes, which have been in general separated into two approaches.22,53 The first approach is to roughen a hydrophobic surface typically consisting of a polymer material with low surface energy, e.g. with methylated and fluorinated carbons groups. The other one is to coat low surface energy molecules such as fluoroalkylsilane on a rough surface of any type of material like ceramics or metals. The former is typically a one-step process and the latter a two-step process. However, some superhydrophobic surfaces have also been processed without deposition of low surface energy molecules on hydrophilic materials.54–57
Surface roughness can vary significantly, depending on the processing methods and processing parameters. A numbers of methods can be used to roughen a surface. Also a wide range of surface roughness (either random or tailored) can have heterogeneous wetting in the Cassie–Baxter state, resulting in a superhydrophobic property. Specific methods and routes to produce superhydrophobic surfaces are considered outside the scope of this review but details are available in many other publications.20,51,58–63
2.5 Typical characterization techniques
The most typical means for superhydrophobicity characterization is to measure the contact angle by a sessile droplet method.64,65 A microliter syringe is used to release a water droplet and an optical system is connected to a computer for data analysis. Typically, 5 droplets are put on different positions on the surface and the average is accepted as the final value. Advancing, receding, sliding, and roll-off angles are obtained using the same system, if the sample holder platform can be tilted while the data is recorded. The sessile droplet method is relatively simple. However the results are dependent on the drop profile, the substrate baseline, contrast, lighting and focusing, and it becomes more complicated if the substrate is non-reflective and macroscopically rough.66
Recently, a new method has been developed to characterize superhydrophobicity based on the ability of water droplets bouncing on the surface, i.e. the number of water bounces.67 It was found that water bouncing only occurred on the surfaces with a water contact angle of over 151°. The authors therefore concluded that a surface capable of achieving one or more bounces could be judged to be superhydrophobic (Fig. 6a). They also found that the number of bounces increased linearly with the water contact angle for surfaces with similar microstructures (Fig. 6b), i.e. a surface which supported 5 bounces was more superhydrophobic than one which supported 3 bounces. They suggested that this new technique provided a universal indication of superhydrophobicity and was able to rule out the disparity in measuring the static water contact angle.67
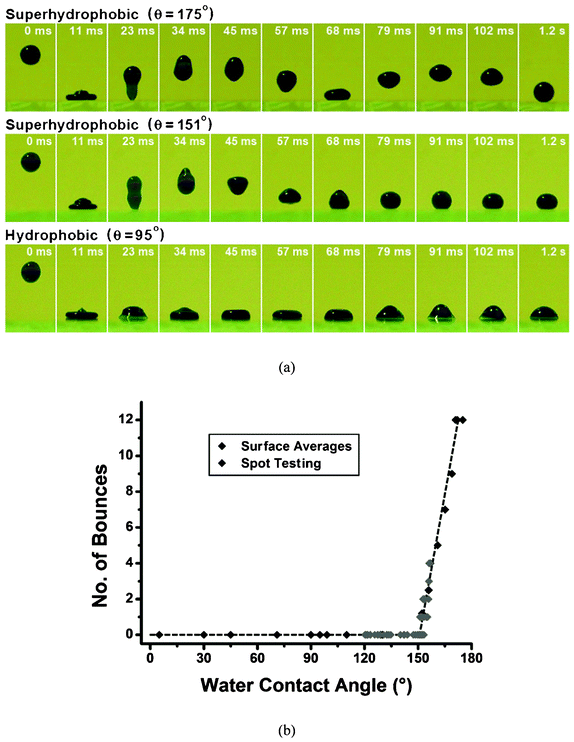 |
| Fig. 6 Series of photographs of an 8 ml methylene blue colored water droplet dropped from a height of 20 mm and bounced on substrates with a range of water contact angles in (a), and the number of bounces on surfaces with a range of hydrophobicity in (b). Reprinted from C. R. Crick and I. P. Parkin, Water droplet bouncing – a definition for superhydrophobic surfaces, Chemical Communications, 2011, 47, 12059–12061, Copyright (2011), with kind permission from Royal Society of Chemistry. | |
Superhydrophobicity is typically characterized in air, and is generally not applicable for submerged systems where the liquid volume is practically infinite. However, underwater superhydrophobicity is relevant in antibacterial applications, when a material surface is immersed in water where bacteria are suspended. One criterion suggested for underwater superhydrophobicity characterization is the area fraction of the solid that is wet at equilibrium (Φe). Thus the goal of designing an underwater superhydrophobic surface has been to make the Φe as small as possible. In principle, underwater superhydrophobicity is achievable with a sufficiently high roughness ratio.68
2.6 Long term durability
Despite the extensive study of superhydrophobic surfaces, challenges still remain in applying them in real applications. As stated, superhydrophobic surfaces require a micro/nano surface roughness and a low surface energy, thus they generally suffer from weak durability due to the mechanically fragile micro/nano structure on the surface and the fast degradation of surface chemistry. Robust superhydrophobic surfaces are generally unattainable at the required micro/nanoscales.69 Any loss of the micro/nano topographical structures on the surfaces will lead to a reduction of its superhydrophobicity. The degradation of the low surface energy layer may lead to the loss of superhydrophobicity even when the surface topographical structure is undamaged. The degradation could be caused by surface contamination63 and/or reactions of the layer with chemicals in the environment.70,71
Although plenty of studies have been carried out developing processes to create superhydrophobic surfaces, research on the durability aspect of superhydrophobic surface only started a few years ago.72 Several concepts to improve the mechanical durability of superhydrophobic surfaces are suggested, for example, by using hierarchical roughness in which robust microscale bumps can provide protection to the more fragile nanoscale roughness,73,74 using composite materials with a hydrophobic polymer in the matrix,75–77 and incorporating self-healing78,79 and photocatalytic functions in superhydrophobic surfaces.72 The uses of hierarchical roughness and composite materials have been currently considered as the most mature concept and other concepts are still in early development stages. It has also been found that, although the hierarchical roughness covered with a low surface energy layer has attracted a lot of attention, the wear out of the low surface energy layer and damage of the nanoscale roughness can result in an increase in the contact angle hysteresis and loss of superhydrophobicity.73,74 Incorporating various nanoparticles in the hydrophobic polymer matrix in composite materials has been demonstrated as a reasonable approach to improve durability.75–77 Despite the great efforts made so far, it is still challenging to obtain mechanically robust and chemically stable and durable superhydrophobic surfaces for demanding applications, and certainly much more effort and breakthrough solutions are required. For many applications where wear resistance is not essential, superhydrophobic surfaces can still be applied to achieve a good performance. For example superhydrophobic surfaces can be applied on the ceilings and walls of hospital buildings to reduce bacterial adhesion. Therefore it is important to understand the durability requirements in targeted applications in order to apply a proper processing method in preparing a suitable superhydrophobic structure.
3 Effect of material properties on bacterial adhesion
Although this review focuses on the reduction of bacterial adhesion via superhydrophobicity, it is important to explain how bacteria interact with materials and how material properties in general affect bacterial adhesion. This section thus identifies the materials properties and summarizes their effects on bacterial adhesion. It is not intended to provide concrete conclusions on the exact influence of a material property on bacterial adhesion, since contradictory results have been observed depending on the particular experimental conditions applied in different studies. Joint influences due to a number of material properties are often seen.
3.1 Bacterial adhesion in general
In general, bacteria are structurally and chemically complex and dynamic to environmental changes.80 They can be classified by their shape or as Gram positive or Gram negative, which refers to a staining procedure, as shown in Fig. 7. For example Staphylococcus epidermidis is a spherical coccus and Gram positive, and Escherichia coli is a cylindrical bacillus and Gram negative.81 The composition of the cell wall of Gram positive and Gram negative bacteria differs considerably.80
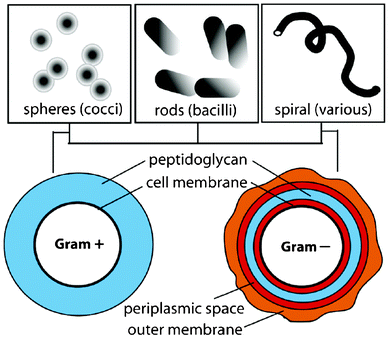 |
| Fig. 7 Illustration of bacterial classification. Reprinted with permission from J. A. Lichter, K. J. V. Vliet and M. F. Rubner, Design of Anti-bacterial Surfaces and Interfaces: Polyelectrolyte Multilayers as a Multifunctional Platform, Macromolecules, 2009, 42, 8573, Copyright (2009) American Chemical Society. | |
Classically, bacterial cells have been treated as inert particles and the bacterial adhesion to a flat surface have been widely described by the DLVO (Derjaguin, Landau, Verwey, Overbeek) theory in which particle adhesion is governed by van der Waals interactions (generally attractive) and repulsive interactions from the electrical double layer of the cell and the surface.82 Bacterial adhesion is generally described by two stages resulting in mature biofilm formation, as illustrated in Fig. 8. Stage I is the initial interaction which is rapid and reversible between the bacterial cell surfaces and the material surfaces, while stage II involves specific and non-specific interactions between proteins on the bacterial surface structures (fimbriae or pilli) and binding molecules on the material surface. Stage II is slowly reversible and often termed as irreversible.81 However, real cell-material interactions are far more complex as they involve highly dynamic cells, the environment with many variables such as temperature, pH, etc., and material surfaces influenced by many different properties. This paper will focus on the effect of material properties on the bacterial adhesion.
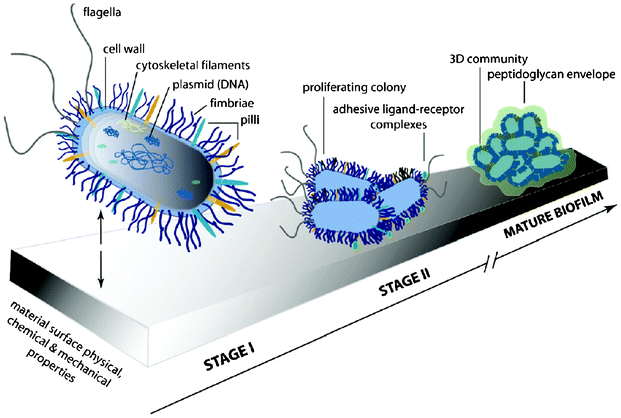 |
| Fig. 8 Illustration of the two stages of bacterial adhesion. Reprinted with permission from J. A. Lichter, K. J. V. Vliet and M. F. Rubner, Design of Anti-bacterial Surfaces and Interfaces: Polyelectrolyte Multilayers as a Multifunctional Platform, Macromolecules, 2009, 42, 8573, Copyright (2009) American Chemical Society. | |
3.2 Effect of surface charge
Surface charge is typically originated from atom substitution in a crystal lattice, lattice defects, broken bonds and the ionization of surface groups. The surface charge affects the distribution of ions in the surrounding interfacial region, resulting in an increased concentration of counter ions (ions of opposite charge to that of the particle) close to the surface. Thus an electrical double layer exists around each particle, and the Z potential is typically measured to indicate the thickness of the electrical double layer.83
The bacterial cell surface charge originates from the dissociation or protonation of carboxyl, phosphate and amino groups.80 Under most physiological conditions, a bacterial cell surface carries a negative charge, with a few exceptions.80,84 Therefore, the bacterial adhesion on negatively charged surfaces is generally reduced as bacteria experience an electric double layer repulsion when approaching the surface. On the contrary, bacterial adhesion tends to increase on positively charged surfaces.85 However, Gottenbos et al.86 found that positive surface charge could also impede bacterial growth, despite the initially promoted adhesion.
3.3 Effect of surface free energy
Bacterial adhesion on surfaces with different surface free energies has been widely studied and it has been proved that the surface free energy can significantly influence bacterial adhesion. A relationship between bacterial adhesion and the surface free energy was reported and a surface free energy between 23 and 30 mN m−1 was related to the lowest bacterial adhesion.87 This is consistent with other reported results. For example, a minimum Escherichia coli adhesion was reported for the surface free energy range between 21 and 29 mN m−1.88 A minimum bioadhesive range and a highest detachment rate of marine biofilms was shown to exist for a surface free energy between 20 and 25 mN m−1.89 A tailoring of a total surface free energy between 20 and 27 mN m−1 and its LW component between 20 and 24 mN m−1 reduced the initial adhesion of Pseudomonas aeruginosa AK1 to inert surfaces.90
It is also reported that bacteria spreading was dependant on the polar component of the surface free energy (γAB). The spreading was low when the polar component was less than 5 mN m−1, while high spreading occurred when the polar component was greater than 15 mN m−1.40 The Lewis acid (electron-acceptor, γ+) and Lewis base (electron-donor, γ−) parameters of surface energy have also been reported to have influence on the growth and attachment of osteoblasts as surfaces with more electron acceptor sites encouraged osteoblastic differentiation.40
3.4 Effect of wettability
A lot of research has also focused on investigating the influence of surface hydrophobicity or hydrophilicity, in another word, the wettability on bacterial adhesion.91–96 Arima and Iwata91 found that bacteria effectively adhered onto polymer surfaces with water contact angles of 40–70°. Also, Lee et al.92 reported that bacteria adhered, spread and grew more on a polyethylene surface with moderate hydrophilicity, with a maximum adhesion of bacteria at a water contact angle of about 57°. Both studies showed a good relationship between wettability and bacterial adhesion. Studies also showed that the hydrophilic surface attracted bacteria like Staphylococcus aureus and Escherichia coli,93 whereas hydrophobic surface attracted Pseudoxanthomonas taiwanensis and Staphylococcus epidermidis and reduced the adherence of bacteria including Deinococcus geothermalis and Meiothermus Silvanus,94Staphylococcus aureus,95 and Streptococcus mutans.96
Surface charge, surface free energy and surface wettability are related and often have joint influence on bacterial adhesion. Besides, they all depend on the surface chemical composition whose effect on bacterial adhesion is typically studied through surface wettability, free energy and charge of the material.
3.5 Effect of surface topography
It was found that irregularities in polymeric surfaces promoted bacterial adhesion and biofilm formation, whereas ultrasmooth surfaces did not favour bacterial adhesion and biofilm formation.97 This was explained by the fact that a rough surface processes a greater surface area with the depressions providing more favourable sites for bacterial colonization. By using natural surfaces the effect of surface topography on biofilm formation and subsequently their fouling in sea water was studied. One example of natural surfaces is the shell of the blue mussel Mytilus galloprovincialis which has a homogenous ripple-like microtextured surface (1–2 μm). It was reported with microtopography that the shell of M. galloprovincialis was fouled by significantly fewer species and had a significantly less total fouling cover than the smooth reference surfaces over a 12 week period in sea water.98 An attachment point theory was later studied to explain the effect of surface topography on bacterial adhesion, the subsequent biofilm formation and fouling. Theoretically, the cell has the highest number of attachment points when it fits within the depressions in the microtextures, while 2 attachment points are the lowest when the cell is slightly larger than the microtexture wavelength.99 In general, the surfaces with a higher number of attachment points will attract more cell attachment. However, the influence of the surface topography addressed here is not the same on superhydrophobic surfaces with Cassie–Baxter wetting schemes. The application of using superhydrophobic surfaces to reduce bacterial adhesion is reviewed in the next section.
3.6 Effect of other material properties
Apart from the above mentioned material properties, the elastic modulus and surface coating thickness were also reported to affect bacterial adhesion. Brady and Singer100 found that the relative bioadhesion of pseudobarnacles on various surfaces was related to (γE)1/2, where γ is the surface energy and E is the elastic modulus or Young's modulus. They also suggested that bacterial adhesion decreased when the coating thickness was increased to 100 μm. A coating thickness of more than 100 μm did not exhibit any major decrease in the adhesion.
4 Recent developments in applying superhydrophobic surfaces to reduce bacterial adhesion
Bacterial adhesion on hydrophobic surfaces has been extensively studied and it has been recently extended to superhydrophobic surfaces. The feasibility of using superhydrophobic surfaces to reduce bacterial adhesion has been investigated by a number of researchers.101–118 This section reviews the superhydrophobic surfaces that have been shown to reduce bacterial adhesion and the mechanisms of how bacterial cells interact with superhydrophobic surfaces, evaluating the current development in applying superhydrophobic surfaces for the reduction of bacterial adhesion.
4.1 Superhydrophobic surfaces to reduce bacterial adhesion
Table 1 summarizes the experimental details and the main findings of the superhydrophobic surfaces that have shown the ability to reduce bacterial adhesion with a couple of exceptions.
Table 1 Summary of the bacterial adhesion experimental details and the main findings
Bacterium tested |
Material and processing of the superhydrophobic surface |
Test period |
Main findings on the superhydrophobic surface |
Ref. |
Staphylococcus aureus
(spherical shape, Gram positive) |
Based on TiO2 nanotubes processed by electrochemical oxidation and finally treated with silane |
2 h and 4 h |
Less adhesion than on hydrophobic and hydrophilic surfaces, scattered distribution, adhesion increased with time |
101
|
Based on fluorinated silica colloids |
1.5 h |
Reduced adhesion compared with reference |
102
|
Based on a silicone elastomer prepared by aerosol assisted chemical vapour deposition |
1 h |
Reduced adhesion compared with an uncoated glass and a dip-coated elastomer hydrophobic glass |
103
|
Superhydrophobic poly(L-lactic acid) surface |
24 h |
No reduced bacterial adhesion
|
106
|
Laser ablated superhydrophobic Ti surface |
18 h |
No reduced bacterial adhesion and bacterial colonization happened
|
105
|
Pseudomonas aeruginosa
(rod shape, Gram negative) |
Based on fluorinated silica colloids |
1.5 h |
Reduced adhesion compared with reference |
102
|
Laser ablated superhydrophobic Ti surface |
18 h |
Nearly no bacterial adhesion |
105
|
Superhydrophobic poly(L-lactic acid) surface |
24 h |
No reduced bacterial adhesion and biofilm formation happened
|
106
|
Escherichia coli
(rod shape, Gram negative) |
polystyrene (PS), polycarbonate (PC) and polyethylene (PE) surfaces induced by shrink |
NA |
2% of bacteria adhered, < 0.1% of bacteria remained after rinsing |
104
|
Based on a silicone elastomer prepared by aerosol assisted chemical vapour deposition |
1 h |
Reduced adhesion compared with an uncoated and a dip-coated elastomer hydrophobic glass |
103
|
Tang et al.101 observed the adhesion of Staphylococcus aureus on surfaces with different wettabilities. The SEM images of the adhesion of Staphylococcus aureus on different surfaces after 2 h and 4 h are shown in Fig. 9. After 2 h, there were Staphylococcus aureus on their TiO2 nanotube based superhydrophobic surface with a water contact angle of 156° compared with those on the hydrophilic surface with a water contact angle of 54° and the hydrophobic surface with a water contact angle of 133°. Furthermore, the Staphylococcus aureus that adhered onto the hydrophobic and superhydrophobic surfaces were more scattered, while the Staphylococcus aureus that adhered onto the hydrophilic surfaces tended to gather. After 4 h, similar trends were found, except that the amount of adhered cells on all three surfaces increased. The authors concluded that although the cells were not totally absent on the superhydrophobic surfaces and the amount of adhered cells increased with time, they were much less in quantity and more scattered than those on the hydrophilic and hydrophobic surfaces and could be easily removed.
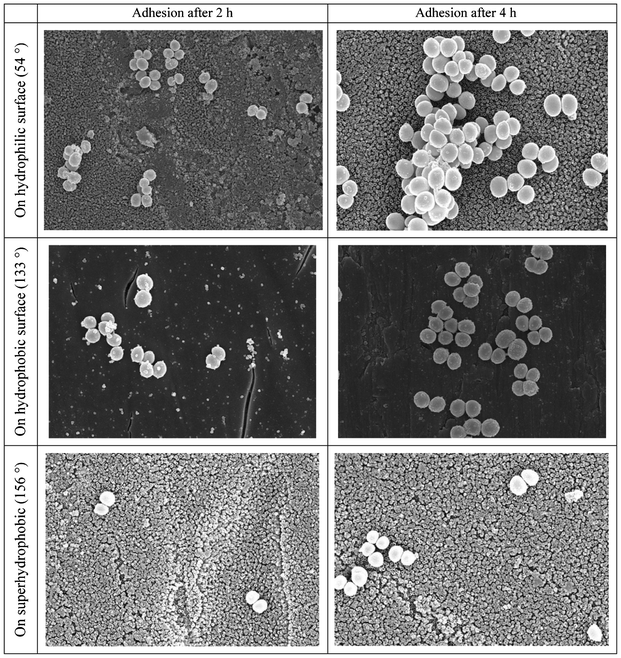 |
| Fig. 9 SEM images of Staphylococcus aureus adhesion after 2 h and 4 h on hydrophilic, hydrophobic and superhydrophobic surfaces with contact angles marked in the parentheses. Reprinted with permission from P. Tang, W. Zhang, Y. Wang, B. Zhang, H. Wang, C. Lin and L. Zhang, Effect of superhydrophobic surface of titanium on Staphyococcus aureus adhesion, Journal of Nanomaterials, 2011, article ID 178921. | |
Privett et al.102 demonstrated that the adhesion of Staphylococcus aureus and Pseudomonas aeruginosa was reduced significantly on the superhydrophobic coating (water contact angle of 167°) obtained from fluorinated silica colloids. Crick et al.103 reported reduced Staphylococcus aureus and Escherichia coli adhesion on their AACVD (aerosol assisted chemical vapour deposition) coated superhydrophobic surface (water contact angel of 165°) compared with an uncoated plain glass (water contact angle of 60°) and a dip-coated elastomer glass (water contact angle of 95°). Furthermore, Freschauf et al.104 found a good prevention of Escherichia coli growth on their shrink-induced superhydrophobic polystyrene (PS), polycarbonate (PC) and polyethylene (PE) surfaces and also demonstrated the effectiveness of rinsing on removing bacterial cells. Only 2% of the initial bacterial cells adhered on the superhydrophobic surface. Rinsing removed nearly all the cells with less than 0.1% of the initial bacterial cells remaining on the surface.
On the other hand, Fadeeva et al.105 reported that Staphylococcus aureus cells were colonized after 18 h on the laser ablated superhydrophobic Ti surface though Pseudomonas aeruginosa cells did not attach to the surface. The authors suggested that the spherical Staphylococcus aureus cells (typically 1 μm in diameter) required a much lower degree of surface contact to adhere compared to the rod-like Pseudomonas aeruginosa cells (typically 1–5 μm long and 0.5–1 μm wide). On the other hand, Sousa et al.106 reported both Staphylococcus aureus and Pseudomonas aeruginosa colonized on their superhydrophobic poly(L-lactic acid) surface after 24 h and Pseudomonas aeruginosa was even able to form a biofilm on the surface. Furthermore, the bacteria were not able to be removed easily by water. However, further discussions on the mechanisms were not given in the paper.
Reducing bacterial adhesion via superhydrophobicity is a relatively new topic and has yet to be studied thoroughly and systematically. The majority of the current research is still focusing on investigating the phenomenon and the potential of applying superhydrophobic surfaces to reduce bacterial adhesion. As shown in Table 1, the tested superhydrophobic surfaces are based on different materials processed by different methods. The only parameter that is constant is the contact angle for water, which is over 150° on all the surfaces. The bacterium types tested are limited to Staphylococcus aureus, Escherichia Coli and Pseudomonas aeruginosa. The bacterial adhesion test procedure also differs from study to study. In some studies a bacterial suspension was flown over the samples while in others the samples were immersed in the bacterial suspension, with the incubation time varying between 1 and 24 h. Therefore, it is not surprising that contradictive results could be obtained as the experimental details could significantly affect the results. In order to fully understand the mechanisms of bacterial adhesion on superhydrophobic surfaces, more bacterium types should be tested and the cell adhesion test procedure should be standardized. On the other hand, more parameters, i.e. surface roughness values, morphology, functional group and the free energy from the superhydrophobic surfaces, should also be considered. The interaction between bacterial cells and superhydrophobic surfaces should be systematically studied. The two-stage bacterial adhesion shown in Fig. 8 is not necessarily applicable to superhydrophobic surfaces. However, despite the variations in these experiments, a strong potential for using a superhydrophobic surface to reduce the initial bacterial adhesion is seen, although further efforts are still required.
4.2 Reducing bacterial adhesion via reduced protein adsorption
Despite the variations in the results achieved in the studies on superhydrophobic surfaces, it has been agreed that reduced protein adsorption plays an important role in reducing bacterial adhesion on surfaces. Bacterial adhesion is mediated by different types of interactions which can be nonspecific or specific, for example through an adsorbed protein film.107 The formation of a protein layer can promote bacterial adhesion and facilitate the formation of a biofilm. It is generally considered that proteins tend to adsorb more favourably onto surfaces with contact angles of 60–90° and on hydrophobic surfaces.108 However, superhydrophobic surfaces have been found to have low protein adsorption and easy protein detachment,109,110 thus resulting in a low bacterial adhesion.108,111,112 For example, Stallard et al.108 reported a much lower adhesion of the bovine fibrinogen (Fg) and bovine serum albumin (BSA) proteins on their atmospheric plasma deposited superhydrophobic fluorinated siloxane coatings, compared with hydrophobic surfaces (see Fig. 10). They also performed bacterial adhesion experiments using Staphylococcus aureus to the surfaces before and after a protein adsorption to identify the effect of an adsorbed protein layer on bacterial adhesion. Staphylococcus aureus is known to bind specifically to Fg. The results in Fig. 11 revealed that a very low amount of Staphylococcus aureus cells attached the superhydrophobic Ti surface after Fg adsorption compared to the plain Ti surface, as well as to the plain Ti surface after Fg adsorption. It also showed that Fg treatment did not increase the amount of attached Staphylococcus aureus cells on the superhydrophobic surface without Fg treatment. This was thought to be due to the lack of an adsorbed Fg protein layer on the superhydrophobic surface even after the Fg treatment. No significant difference in the adhesion between the plain Ti and Fg treated plain Ti surfaces was observed though one could expect the Fg treated plain Ti surface would attract more bacterial cells than the non-treated plain Ti surface. However, the authors did not offer a discussion on this.
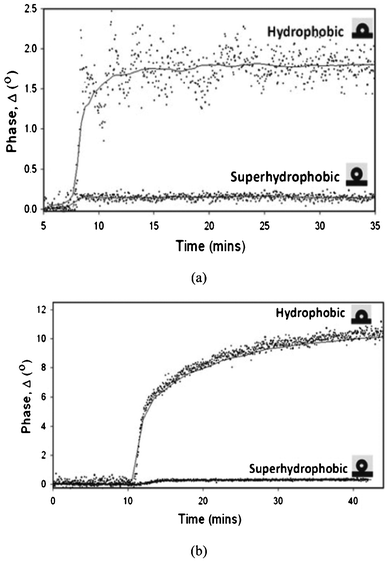 |
| Fig. 10 Adsorption profiles of BSA (a) and Fg (b) on hydrophobic and superhydrophobic surfaces. Reprinted with permission from C. P. Stallard, K. A. McDonnell, O. D. Onayemi, J. P. O'Gara and D. P. Dowling, Evaluation of protein adsorption on atmosphere plasma deposited coatings exhibiting superhydrophilic to superhydrophobic properties, Biointerphases, 2012, 7, 31. | |
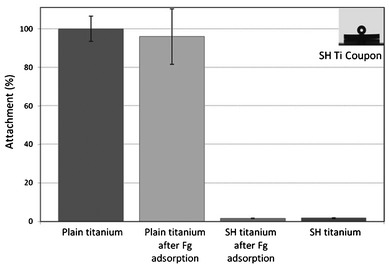 |
| Fig. 11 Attachment of Staphylococcus aureus cells to Fg treated and untreated plain Ti and fluorosiloxane coated superhydrophobic (SH) Ti surface. Reprinted with permission from C. P. Stallard, K. A. McDonnell, O. D. Onayemi, J. P. O'Gara and D. P. Dowling, Evaluation of protein adsorption on atmosphere plasma deposited coatings exhibiting superhydrophilic to superhydrophobic properties, Biointerphases, 2012, 7, 31. | |
4.3 Bacterial adhesion patterns on superhydrophobic surfaces
Although most of the reduced bacterial adhesion on a superhydrophobic surface is shown to be based on a CPU count (bacterial colony producing units) of the adhered cells, some have studied the bacterial adhesion patterns and its possible mechanism on superhydrophobic surfaces. Truong et al.113 tested the bacterial adhesion on laser ablated superhydrophobic titanium surfaces using strains of Staphylococcus aureus CIP 65.8T, Staphylococcus aureus ATCC 25923, Staphylococcus epidermidis ATCC 14990T and Planococcus maritimus KMM 3738. The results showed that each strain preferentially attached to the crevices between the topographical features of the superhydrophobic surface, while the upper regions of the topographical features were cell-free, as shown in Fig. 12.
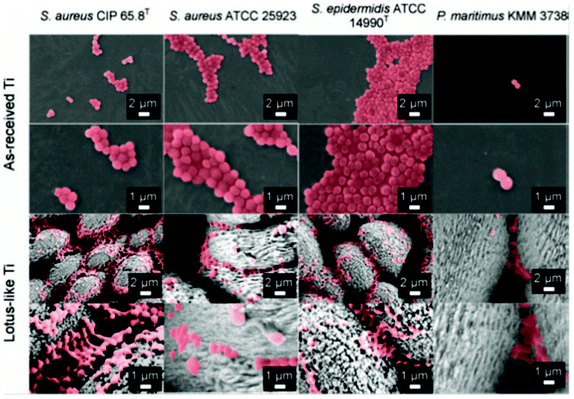 |
| Fig. 12 SEM images of bacterial adhesion patterns on superhydrophobic Lotus-like Ti surfaces and as-received reference Ti surfaces after incubation for 18 h. Reprinted by kind permission of Taylor & Francis Ltd, from Air-directed attachment of coccoid bacteria to the surface of superhydrophobic lotus-like titanium, V. K. Truong, H. K. Webb, E. Fadeeva, B. N. Chichkov, A. H. F. Wu, R. Lamb, J. Y. Wang, R. J. Crawford and E. P. Ivanova, Biofouling: the Journal of Bioadhesion and Biofilm Research, 2012, 28, 539–550. | |
A mechanism has also been proposed in Fig. 13 where the cells were unable to cross the air–water interface and then began to accumulate in the tri-phase interface that provided the best shelter from water turbulence. Subsequently the bacterial cells slid across the nanoscale bubbles trapped within the nanoscale topographical features. With this mechanism, it can be predicted that the bacterial adhesion would be increased over time due to the replacement of trapped air. It also provides an explanation to the findings reported in reference 101.
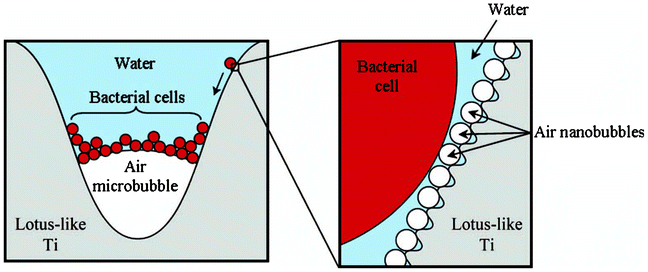 |
| Fig. 13 Proposed mechanism by which bacterial cells accumulate at the tri-phase interface on immersed superhydrophobic Ti surfaces. Reprinted by kind permission of Taylor & Francis Ltd, from Air-directed attachment of coccoid bacteria to the surface of superhydrophobic lotus-like titanium, V. K. Truong, H. K. Webb, E. Fadeeva, B. N. Chichkov, A. H. F. Wu, R. Lamb, J. Y. Wang, R. J. Crawford and E. P. Ivanova, Biofouling: the Journal of Bioadhesion and Biofilm Research, 2012, 28, 539–550. | |
4.4 Bacterial adhesion on a wet superhydrophobic surface
Though it is commonly reported that superhydrophobicity and its capability to reduce bacterial adhesion will be lost under submerged conditions over time after the trapped air is completely excluded,114,115 the naturally superhydrophobic Taro (Colocasia esculenta) leaf has been shown to be highly resistant to bacterial adhesion even in completely wet conditions simulated by treating the leaves with 95% ethanol.116Fig. 14 shows the Pseudomonas aeruginosa adhesion on a Taro leaf under non-wet and wet conditions after 23 h, together with the morphology of a Taro leaf with the corresponding adhesion force on its nanostructures. Both on the wet and non-wet leaf surface, cells adhered mostly on the boundary between epidermal cell units, displaying circular patterns in Fig. 14a and 14b. The authors suggested that the pattern was attributed to the difference in the surface topographical nanostructures in different densities. The boundary area had a low density of nanostructures with a high adhesion force and thus high cell adhesion, while the edge area of epidermal units had high density of nanostructures with low adhesion force and therefore low cell adhesion (see Fig. 14c). As a conclusion, the authors suggested that properly designed surface nanostructures might be able to reduce bacterial adhesion even in completely wet submerged conditions.
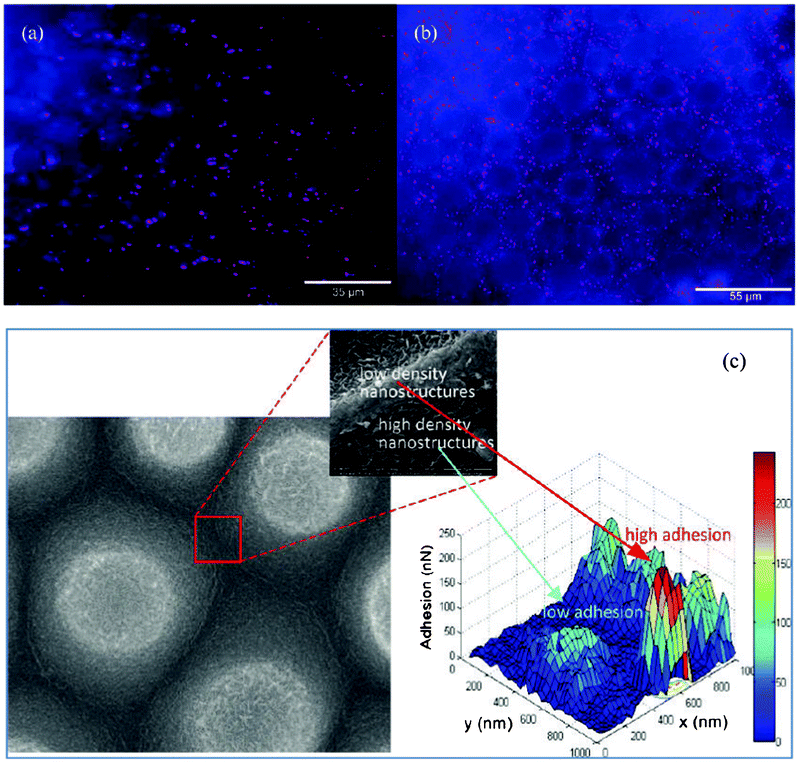 |
| Fig. 14
Pseudomonas aeruginosa adhesion on a Taro leaf under non-wet conditions in (a) and wet conditions in (b). SEM image of a Taro leaf (left in c) and the corresponding adhesion force map (right in c). Reprinted with kind permission from J. Ma, Y. Sun, K. Gleichauf, J. Lou and Q. Li, Nanostructure on Taro leaves fouling by colloids and bacteria under submerged conditions, Langmuir, 2011, 27, 10035–10040, Copyright (2011) American Chemical Society. | |
4.5 Other relevant applications
Superhydrophobic surfaces have also been found to have increased resistance to the microbial-induced corrosion (MIC) and fouling in sea water due to its capability to reduce bacterial adhesion.115,117,118 Zhang et al.115 reported that their superhydrophobic surface had a promising anti-fouling property in the short term. However, after long term exposure to sea water, the superhydrophobic film lost its anti-fouling property. Liu et al.117 found that the marine bacterium Vibrio natriegens had only a weak attachment to a superhydrophobic aluminium surface, leading to a significant reduction in the corrosion rate of aluminium due to the lack of Vibrio natriegens. Mahalakshmi et al.118 tested their superhydrophobic titanium surface in seawater and found out that microbes did not attach on the surface and the corrosion resistance of titanium in seawater was significantly increased.
4.6 Hybrid solutions in developing antibacterial materials
Although the concept of using superhydrophobicity against bacterial adhesion is green and environmental friendly, the efficiency is rather low, as indicated from the phenomenon that bacteria will adhere to the superhydrophobic surface over time after the surface become fully wet and the entrapped air is excluded. As aforementioned in the introduction, the use of antibacterial metals and photocatalytic materials form the other parts of the new strategies in developing antibacterial materials besides superhydrophobicity. Attempts at developing hybrid antibacterial materials by combining superhydrophobicity with antibacterial metals or with photocatalysis have been made in order to improve the antibacterial performance while maintaining the green concept of preventing bacterial adhesion in the first place. The efforts of incorporating antibacterial metals such as Ag119,120 and Cu17 with superhydrophobicity have been more successful than combining photocatalysis with superhydrophobicity. Tang et al.121 combined superhydrophobicity with photocatalysis by coating a TiO2 surface with fluorosilane to see the antibacterial effect. Unfortunately the thin surface silane layer would largely stop the photocatalysis. Superhydrophobic ZnO has also been fabricated122 but antibacterial testing was hardly reported.
5 Concluding remarks
In general, materials properties such as surface energy, wettability (hydrophobicity and hydrophilicity), surface charge, surface chemical composition, surface topography, surface elastic modulus and coating thickness were found to have an influence or a joint influence on the bacterial adhesion on surfaces. Mild hydrophobicity does not always reduce bacterial adhesion.
Different from hydrophobic surfaces, superhydrophobic surfaces have shown a promising capability to reduce bacterial adhesion together with an easy removal of bacteria cells, enabling the superhydrophobic surface to be a new strategy in the antibacterial design. The reduced bacterial adhesion on superhydrophobic surfaces was found to result from the reduced protein adsorption and the entrapped air layer between the bacteria cells and the surface. There are some studies that have investigated the interaction of bacteria cells with superhydrophobic surfaces and speculated the possible mechanism. However, the study of bacterial adhesion on superhydrophobic surfaces is not yet sufficiently extensive and systematic. The tested bacteria are rather limited, most commonly with Staphylococcus aureus, Escherichia Coli and Pseudomonas aeruginosa. As bacterial properties play significant roles in the bacteria–material interaction, more bacteria types should be characterized to identify the interaction mechanism on superhydrophobic surfaces. The experimental procedures in the cell adhesion test also differ considerably with many variables (e.g. incubation time from 1 h to 24 h) and thus make it very difficult to compare results to draw common conclusions. In addition to water contact angles, more parameters e.g. surface roughness values, morphology, functional group and free energy from the superhydrophobic surfaces should be studied to see their effect on bacterial adhesion. On the other hand, superhydrophobic surfaces are a great concept to change surface properties but unfortunately suffer from a weak durability due to its micro/nano surface structures and fast degradation of surface chemistry. Although initial progress has been reported, more efforts are required to improve the durability of superhydrophobic surfaces.
Certain types of superhydrophobic surfaces have proved to be effective in reducing the bacterial adhesion for up to 24 h. Over time bacteria cells will colonize on superhydrophobic surfaces after the surfaces have become fully wet and the entrapped air is excluded. However, there is one exciting study existing on the naturally superhydrophobic Taro leaf showing the leaf is still effective against bacteria even under wet conditions. With a further development in the design of the surface structures it may be possible to eliminate the adhesion of bacteria on superhydrophobic surfaces even under wet conditions. Efforts at developing hybrid antibacterial materials by combining superhydrophobicity with antibacterial metals or with photocatalysis have also been attempted. However, the combination of superhydrophobicity, photocatalysis and antibacterial metallic materials still require a much longer-term development for success in antibacterial applications.
Acknowledgements
The financial support from the Finnish Funding Agency for Technology and Innovation (Tekes) and the Centennial Foundation from the Technology Industries of Finland is acknowledged. The authors are also thankful to Prof. Robert Wood for his help in arranging Dr Zhang's research visit at nCATS.
References
- J. A. Lichter, K. J. V. Vliet and M. Rubner, Design of antibacterial surfaces and interfaces: polyelectrolyte multilayers as a multifunctional platform, Macromolecules, 2009, 42, 8573–8586 CrossRef CAS
.
- K. Poole, Mechanisms of bacterial biocide and antibiotic resistance, J. Appl. Microbiol., 2002, 92, 55S–64S CrossRef
.
- G. McDonnell and A. D. Russell, Antiseptics and disinfectants: activity, action and resistance, Clin. Microbiol. Rev., 1999, 12, 147–179 CAS
.
- A. D. Russell, Bacterial resistance to disinfectants: present knowledge and future problems, J. Hosp. Infect., 1999, 43 (Supp.), S453–S464 Search PubMed
.
- A. D. Russell, Antibiotic and biocide resistance in bacteria: introduction, J. Appl. Microbiol., 2002, 92, S1–S3 CrossRef
.
- M. Rai, A. Yadav and A. Gade, Silver nanoparticles as a new generation of antimicrobials, Biotechnol. Adv., 2009, 27, 76–83 CrossRef CAS
.
- M. T. Hsiao, S. F. Chen, D. B. Shieh and C. S. Yeh, One-pot synthesis of hollow Au3Cu1 spherical-like and biomineral botallackite Cu2(OH)3Cl flowerlike architectures exhibiting antimicrobial activity, J. Phys. Chem. B, 2006, 110, 205–210 CrossRef CAS
.
- M. Yasuyuki, K. Kunihiro, S. Kurissery, N. Kanavillil, Y. Sato and Y. Kikuchi, Antibacterial properties of nine pure metals: a laboratory study using Staphylococcus aureus and Escherichia coli, Biofouling, 2010, 26, 851–858 CrossRef CAS
.
- C. M. Jones and E. M. V. Hoek, A review of the antibacterial effects of silver nanomaterials and potential implications for human health and the environment, J. Nanopart. Res., 2010, 12, 1531–1551 CrossRef
.
- A. Gupta and S. Silver, Silver as a biocide: will resistance become a problem, Nat. Biotechnol., 1998, 16, 888 CrossRef CAS
.
- G. Fu, P. S. Vary and C. T. Lin, Anatase TiO2 nanocomposites for antimicrobial coatings, J. Phys. Chem. B, 2005, 109, 8889–8898 CrossRef CAS
.
- P. C. Maness, S. Smolinski, D. M. Blake, Z. Huang, E. J. Wolfrum and W. A. Jacoby, Bactericidal activity of photocatalytic TiO2 reaction: toward an understanding of its killing mechanism, Appl. Environ. Microbiol., 1999, 65, 4094–4098 CAS
.
- J. A. Ibanez, M. I. Litter and R. A. Pizarro, Photocatalytic bactericidal effect of TiO2 on Enterobacter cloacae: comparative study with other gram (-) bacteria, J. Photochem. Photobiol., A, 2003, 157, 81–85 CrossRef CAS
.
- K. Hashimoto, H. Irie and A. Fujishima, TiO2 photocatalysis: a historical overview and future prospects, Jpn. J. Appl. Phys., 2005, 44, 8269–8285 CrossRef CAS
.
- S. In, A. Orlov, R. Berg, F. Garcia, S. Pedrosa-Jimenez, M. S. Tikhov, D. S. Wright and M. Lambert, Effective visible light-activated B-doped and B,N-codoped TiO2 photocatalysis, J. Am. Chem. Soc., 2007, 129, 13790–13791 CrossRef CAS
.
- J. Genzer and K. Efimenko, Recent developments in superhydrophobic surfaces and their relevance to marine fouling: a review, Biofouling, 2006, 22, 339–360 CrossRef CAS
.
- A. Berendjchi, R. Khajavi and M. E. Yazdanshenas, Fabrication of superhydrophobic and antibacterial surface on cotton fabric by doped silica-based sols with nanoparticles of copper, Nanoscale Res. Lett., 2011, 6, 594 CrossRef
.
- B. J. Privett, J. Youn, S. A. Hong, J. Lee, J. Han, J. H. Shin and M. H. Schoenfisch, Antibacterial fluorinated silica colloid superhydrophobic surfaces, Langmuir, 2011, 27, 9597–9601 CrossRef CAS
.
- C. R. Crick, S. Ismail, J. Pratten and I. P. Parkin, An investigation into bacterial attachment to an elastomeric superhydrophobic surface prepared via aerosol assisted deposition, Thin Solid Films, 2011, 519, 3722–3727 CrossRef CAS
.
- Y. Y. Yan, N. Gao and W. Barthlott, Mimicking natural superhydrophobic surfaces and grasping the wetting process: A review on recent progress in preparing superhydrophobic surfaces, Adv. Colloid
Interface Sci., 2011, 169, 80–105 CrossRef CAS
.
- C. Neinhuis and W. Barthlott, Characterization and distribution of water-repellent, self-cleaning plant surfaces, Ann. Bot., 1997, 79, 667–677 CrossRef
.
- L. Feng, S. Li, Y. Li, H. Li, L. Zhang, J. Zhai, Y. Song, B. Liu, L. Jiang and D. Zhu, Super-hydrophobic surfaces: from natural to artificial, Adv. Mater., 2002, 14, 1857–1860 CrossRef CAS
.
- T. Young, An essay on the cohesion of fluids, Philos. Trans. R. Soc. London, 1805, 95, 65–87 CrossRef
.
- A. Nakajima, Design of hydrophobic surfaces for liquid droplet control, NPG Asia Mater., 2011, 3, 49–56 CrossRef
.
-
M. Nosonovsky and B. Bhushan, Multiscale dissipative mechanisms and hierarchical surfaces: friction, superhydrophobicity and biomimetics, Berlin,Springer, 2008 Search PubMed
.
- R. N. Wenzel, Resistance of solid surfaces to wetting by water, Ind. Eng. Chem., 1936, 28, 988–994 CrossRef CAS
.
- A. Marmur, Super-hydrophobicity fundamentals: implications to biofouling prevention, Biofouling, 2006, 22, 107–115 CrossRef CAS
.
- D. Quere, Rough ideas on wetting, Phys. A, 2002, 313, 32–46 CrossRef CAS
.
- A. B. D. Cassie and S. Baxter, Wettability of porous surfaces, Trans. Faraday Soc., 1944, 40, 546–550 RSC
.
- A. Marmur, The Lotus effect: superhydrophobicity and metastability, Langmuir, 2004, 20, 3517–3519 CrossRef CAS
.
- K. Tadanaga, J. Morinaga, A. Matsuda and T. Minami, Superhydrophobic-superhydrophilic micropatterning on flowerlike alumina coating film by the sol–gel method, Chem. Mater., 1999, 12, 590–592 CrossRef
.
- B. Bhushan, M. Nosonovsky and Y. C. Jung, Towards optimization of patterned superhydrophobic surfaces, J. R. Soc. Interface, 2007, 4, 643–648 CrossRef CAS
.
- B. Bhushan and Y. C. Jung, Wetting study of patterned surfaces for superhydrophobicity, Ultramicroscopy, 2007, 107, 1033–1041 CrossRef CAS
.
- B. Balu, V. Breedveld and D. Hess, Febrication of “roll-off” and “sticky” superhydrophobic cellulose surfaces via plasma processing, Langmuir, 2008, 24, 4785–4790 CrossRef CAS
.
- M. Miwa, A. Nakajima, A. Fujishima, K. Hashimoto and T. Watanabe, Effects of the surface roughness on sliding angles of water droplets on superhydrophobic surfaces, Langmuir, 2000, 16, 5754–5760 CrossRef CAS
.
- D. K. Owens and R. C. Wendt, Estimation of the surface free energy of polymers, J. Appl. Polym. Sci., 1969, 13, 1741–1747 CrossRef CAS
.
- Q. Zhao, Y. Liu and E. W. Abel, Effect of temperature on the surface free energy of amorphous carbon films, J. Colloid Interface Sci., 2004, 280, 174–183 CrossRef CAS
.
- C. J. van Oss, M. K. Chaudhury and R. J. Good, Interfacial Lifshitz-van der Waals and polar interactions in macroscopic systems, Chem. Rev., 1988, 88, 927–941 CrossRef CAS
.
- T. Nishino, M. Meguro, K. Nakamae, M. Matsushita and Y. Ueda, The lowest surface free energy based on -CF3 alignment, Langmuir, 1999, 15, 4321–4323 CrossRef CAS
.
- E. M. Harnett, J. Alderman and T. Wood, The surface energy of various biomaterials coated with adhesion molecules used in cell culture, Colloids Surf., B, 2007, 55, 90–97 CrossRef CAS
.
- W. Barthlott and C. Neinhuis, Purity of the sacred lotus, or escape from contamination in biological surfaces, Planta, 1997, 202, 1–8 CrossRef CAS
.
- X. Zhang, X. Liu, J. Laakso, E. Levänen and T. Mäntylä, Easy-to-clean property and durability of superhydrophobic flaky γ-alumina coating on stainless steel in field test at a paper machine, Appl. Surf. Sci., 2012, 258, 3102–3108 CrossRef CAS
.
- X. Zhang, M. Järn, J. Peltonen, V. Pore, T. Vuorinen, E. Levänen and T. Mäntylä, Analysis of roughness parameters to specify superhydrophobic antireflective boehmite films made by the sol–gel process, J. Eur. Ceram. Soc., 2008, 28, 2177–2181 CrossRef CAS
.
- L. Zhang, Y. Li, J. Sun and J. Shen, Layer-by-layer fabrication of broad-band superhydrophobic antireflection coatings in near-infrared region, J. Colloid Interface Sci., 2008, 319, 302–308 CrossRef CAS
.
- L. Gao, A. K. Jones, V. K. Sikka, J. Wu and D. Gao, Anti-Icing superhydrophobic coatings, Langmuir, 2009, 25, 12444–12448 CrossRef
.
- L. Mishchenko, B. Hatton, V. Bahadur, J. A. Taylor, T. Krupenki and J. Aizenberg, Design of ice free nanostructured surfaces based on repulsion of impacting water droplets, ACS Nano, 2010, 4, 7699–7707 CrossRef CAS
.
- A. J. Meuler, G. H. McKinley and R. E. Cohen, Exploiting topographical texture to impart icephobicity, ACS Nano, 2010, 4, 7048–7052 CrossRef CAS
.
- R. Truesdell, A. Mammoli, P. Vorobieff, P. van Swol and C. J. Brinker, Drag reduction on a patterned superhydrophobic surface, Phys. Rev. Lett., 2006, 97, 044504 CrossRef
.
- R. J. Daniello, N. E. Waterhouse and J. P. Rothstein, Drag reduction in turbulent flows over superhydrophobic surfaces, Phys. Fluids, 2009, 21, 085103 CrossRef
.
- M. Nosonovsky and B. Bhushan, Roughness-induced superhydrophobicity: a way to design non-adhesive surfaces, J. Phys.: Condens. Matter, 2008, 20, 225009 CrossRef
.
- Z. Guo, W. Liu and B. Su, Superhydrophobic surfaces: from natural to biomimetic to functional, J. Colloid Interface Sci., 2011, 353, 335–355 CrossRef CAS
.
- T. Verho, J. T. Korhonen, L. Sainiemi, V. Jokinen, C. Bower, K. Franze, S. Franssila, P. Andrew, O. Ikkala and R. H. A. Ras, Reversible switching between superhydrophobic states on a hierarchically structured surface, Proc. Natl. Acad. Sci. U. S. A., 2012, 109, 10213 CrossRef
.
- H. M. Shang, Y. Wang, S. J. Limmer, T. P. Chou, K. Takahashi and G. Z. Cao, Optically transparent superhydrophobic silica-based films, Thin Solid Films, 2005, 472, 37–43 CrossRef CAS
.
- A. Tuteja, W. Choi, M. Ma, J. M. Mabry, S. A. Mazzelle, G. C. Rutledge, G. H. McKinley and R. E. Cohen, Designing superoleophobic surfaces, Science, 2007, 318, 1618–1622 CrossRef CAS
.
- A. Tuteja, W. Choi, G. H. McKinley, R. E. Cohen and M. F. Rubner, Design parameters for superhydrophobicity and superoleophobicity, MRS Bull., 2008, 33, 752–758 CrossRef CAS
.
- L. Feng, Z. Yang, J. Zhai, Y. Song, B. Liu, Y. Ma, Z. Yang, L. Jiang and D. Zhu, Superhydrophobicity of nanostructured carbon films in a wide range of pH values, Angew. Chem., Int. Ed., 2003, 42, 4217–4220 CrossRef CAS
.
- L. Feng, Y. Song, J. Zhai, B. Liu, J. Xu, L. Jiang and D. Zhu, Creation of a superhydrophobic surface from an amphiphilic polymer, Angew. Chem., Int. Ed., 2003, 42, 800–802 CrossRef CAS
.
- L. Feng, S. Li, H. Li, J. Zhai, Y. Song, L. Jiang and D. Zhu, Super-hydrophobic surface of aligned polyacrylonitrile nanofibers, Angew. Chem., Int. Ed., 2002, 41, 1221–1223 CrossRef CAS
.
- L. Jiang, Y. Zhao and J. Zhai, A lotus-leaf-like superhydrophobic surface: a porous microsphere/nanofiber
composite film prepared by electrohydrodynamics, Angew. Chem., Int. Ed., 2004, 43, 4338–4341 CrossRef CAS
.
- S. Nishimoto and B. Bhushan, Bioinspired self-cleaning surfaces with superhydrophobicity, superoleophobicity, and superhydrophilicity, RSC Adv., 2013, 3, 671–690 RSC
.
- S. S. Latthe, A. B. Gurav, C. S. Maruti and R. S. Vhatkar, Recent progress in preparation of superhydrophobic surfaces: a review, J. Surf. Eng. Mater. Adv. Technol., 2012, 2, 76–94 CAS
.
- Y. K. Lai, Z. Chen and C. J. Lin, Recent progress on the superhydrophobic surfaces with special adhesion: from natural to biomimetic to functional, J. Nanoeng. Nanomanuf., 2011, 1, 18–34 CrossRef CAS
.
- X. M. Li, D. Reinhoudt and M. Crego-Calama, What do we need for a superhydrophobic surface? A review on the recent progress in the preparation of superhydrophobic surfaces, Chem. Soc. Rev., 2007, 36, 1350–1368 RSC
.
- X. Zhang, M. Honkanen, M. Järn, J. Peltonen, V. Pore, E. Levänen and T. Mäntylä, Thermal stability of the structural features in the superhydrophobic boehmite films on austenitic stainless steel, Appl. Surf. Sci., 2008, 254, 5129–5133 CrossRef CAS
.
- X. Zhang, M. Honkanen, V. Pore, E. Levänen and T. Mäntylä, Effect of heat-treating gel films on the formation of superhydrophobic boehmite flaky structures on austenitic stainless steel, Ceram. Int., 2009, 35, 1559–1564 CrossRef CAS
.
- J. Zimmermann, S. Seeger and F. A. Reifler, Water shedding angle: a new technique to evaluate the water-repellent properties of superhydrophobic surfaces, Text. Res. J., 2009, 79, 1565–1570 CrossRef CAS
.
- C. R. Crick and I. P. Parkin, Water droplet bouncing – a definition for superhydrophobic surfaces, Chem. Commun., 2011, 47, 12059–12061 RSC
.
- A. Marmur, Underwater superhydrophobicity: theoretical feasibility, Langmuir, 2006, 22, 1400–1402 CrossRef CAS
.
- J. P. Youngblood and N. R. Sottos, Bioinspirited Materials for self-cleaning and self-healing, MRS Bull., 2008, 33, 732–738 CrossRef CAS
.
- J. Zimmermann, G. R. J. Artus and S. Seeger, Long term studies on the chemical stability of a superhydrophobic silicone nanofilament coating, Appl. Surf. Sci., 2007, 253, 5972–5979 CrossRef CAS
.
- L. Boinovich, A. M. Emelyanenko and A. S. Pashinin, Analysis of long term durability of superhydrophobic properties under continuous contact with water, ACS Appl. Mater. Interfaces, 2010, 2, 1754–1758 CAS
.
- T. Verho, C. Bower, P. Andrew, S. Franssila, O. Ikkala and R. H. A. Ras, Mechanically durable superhydrophobic surfaces, Adv. Mater., 2011, 23, 673–678 CrossRef CAS
.
- Y. Xiu, Y. Liu, B. Balu, D. W. Hess and C. Wong, Robust superhydrophobic surfaces prepared with epoxy resin and silica nanoparticles, IEEE Trans. Compon. Packag. Manuf. Technol., 2012, 2, 395–401 CrossRef CAS
.
- Y. Xiu, Y. Liu, D. W. Hess and C. P. Wong, Mechanically robust superhydrophobicity on hierarchically structured Si surfaces, Nanotechnology, 2010, 21, 155705 CrossRef
.
- P. Pareo, G. L. D. Gregorio, M. Manca, M. S. Pianesi, L. D. Marco, F. Cavallaro, M. Mari, S. Pappada, G. Ciccarella and G. Gigli, Ultra lightweight PMMA-based composite plates with robust superhydrophobic surfaces, J. Colloid Interface Sci., 2011, 363, 668–675 CrossRef CAS
.
- H. Zhou, H. Wang, H. Niu, A. Gestos, X. Wang and T. Lin, Fluoroalkyl silane modified silicone rubber/nanoparticles composite: a superdurable, robust superhydrophobic fabric coating, Adv. Mater., 2012, 24, 2409–2412 CrossRef CAS
.
- X. Zhu, Z. Zhang, X. Men, J. Yang, K. Wang, X. Xu, X. Zhou and Q. Xue, Robust superhydrophobic surfaces with mechanical durability and easy repairability, J. Mater. Chem., 2011, 21, 15793–15797 RSC
.
- Y. Li, L. Li and J. Sun, Bioinspirated slef-healing superhydrophobic coatings, Angew. Chem., 2010, 122, 6265–6269 CrossRef
.
- H. Wang, Y. Xue, J. Ding, L. Feng, X. Wang and T. Lin, Durable, self-healing superhydrophobic and superoleophobic surfaces from fluorinated-decyl polyhedral oligomeric silsesquioxane and hydrolysed fluorinated alkyl silane, Angew. Chem., Int. Ed., 2011, 50, 11433–11436 CrossRef CAS
.
- A. T. Poortinga, R. Bos, W. Norde and H. J. Busscher, Electric double layer interactions in bacterial adhesion to surfaces, Surf. Sci. Rep., 2002, 47, 1–32 CrossRef CAS
.
- J. A. Lichter, K. J. V. Vliet and M. F. Rubner, Design of anti-bacterial surfaces and interfaces: polyelectrolyte multilayers as a multifunctional platform, Macromolecules, 2009, 42, 8573 CrossRef CAS
.
- M. Hermansson, The DLVO theory in microbial adhesion, Colloids Surf., B, 1999, 14, 105–119 CrossRef CAS
.
- Zeta potential an introduction in 30 min, Zetasizer nano series technical note, Malvern Instruments, http://www.malvern.com/.
- B. A. Jucker, H. Harms and A. J. Zehnder, Adhesion of the positively charged bacterium Stenotrophomonas (Xanthomonas) maltophilia 70401 to galss and Teflon, J. Bacteriol., 1996, 178, 5472–5479 CAS
.
- M. Kiremitci-Gumustederelioglu and A. Pesmen, Microbial adhesion to ionogenic PHEMA, PU abd PP implants, Biomaterials, 1996, 17, 443–449 CrossRef
.
- B. Gottenbos, H. C. Van Der Mei and H. J. Busscher, Initial adhesion and surface growth of Pseudomonas aeruginosa on negatively and positively charged Poly(methacrylates), J. Mater. Sci.: Mater. Med., 1999, 10, 853–859 CrossRef CAS
.
- Q. Zhao, S. Wang and H. M. Steinhagen, Tailored surface free energy of membrane diffusers to minimize microbial adhesion, Appl. Surf. Sci., 2004, 230, 371–378 CrossRef CAS
.
-
R. E. Baier, Substrata influences on the adhesion of microorganisms and their resultant new surface properties, ed. G. Bitton, K. S. Marshall , in Adsorption of micro-organisms to surface, Wiley/Interscience, New York, 1980, pp. 59–104 Search PubMed
.
- K. Becker, Detachment studies on microfouling in natural biofilms on substrata with different surface tensions, Int. Biodeterior. Biodegrad., 1998, 41, 93–100 CrossRef
.
- C. I. Pereni, Q. Zhao, Y. Liu and E. Abel, Surface free energy effect on bacterial retention, Colloids Surf., B, 2006, 48, 143–147 CrossRef CAS
.
- Y. Arima and H. Iwata, Effect of wettability and surface functional groups on protein adsorption and cell adhesion using well-defined mixed self-assembled monolayers, Biomaterials, 2007, 28, 3074–3082 CrossRef CAS
.
- J. H. Lee, G. Khang, J. W. Lee and H. B. Lee, Interaction of different types of cells of polymer surfaces with wettability gradient, J. Colloid Interface Sci., 1998, 205, 323–330 CrossRef CAS
.
- J. Li and W. Zhang, Bacterial behaviours on polymer surfaces with organic and inorganic antimicrobial compounds, J. Biomed. Mater. Res., Part A, 2008, 88A, 448–453 Search PubMed
.
- M. Raulio, M. Järn, J. Ahola, J. Peltonen, J. B. Rosenholm, S. Tervakangas, J. Kolehmainen, T. Ruokolainen, P. Narko and M. Salkinoja-Salonen, Microbe-repelling coated stainless steel analysed by field emission scanning electron microscopic and physicochemical methods, J. Ind. Microbiol. Biotechnol., 2008, 35, 751–760 CrossRef CAS
.
- H. Yang and Y. Deng, Preparation and physical properties of superhydrophobic papers, J. Colloid Interface Sci., 2008, 325, 588–593 CrossRef CAS
.
- A. Okada, T. Nikaido, M. Ikeda, K. Okada, J. Yamauchi, R. M. Foxton, H. Sawada, J. Tagami and K. Matin, Inhibition of biofilm formation using newly developed coating materials with self-cleaning properties, Dent. Mater. J., 2008, 27, 565–572 CrossRef CAS
.
- T. R. Scheuerman, A. K. Camper and M. A. Hamilton, Effects of substratum topography on bacterial adhesion, J. Colloid Interface Sci., 1998, 208, 23–33 CrossRef CAS
.
- A. J. Scardino and R. de NYS, Fouling deterrence on the bivalve shell Mytilus galloprovincialis: a physical phenomenon, Biofouling, 2004, 20, 249–257 CrossRef CAS
.
- A. J. Scardino, E. Harvey and R. de NYS, Testing attachment point theory: diatom attachment on microtextured polyimide biomimics, Biofouling, 2006, 22, 55–60 CrossRef CAS
.
- R. F. Brady and I. L. Singer, Mechanical factors favouring release from fouling release coating, Biofouling, 2000, 15, 73–81 CrossRef CAS
.
- P. Tang, W. Zhang, Y. Wang, B. Zhang, H. Wang, C. Lin and L. Zhang, Effect of superhydrophobic surface of titanium on Staphyococcus aureus adhesion, J. Nanomater., 2011 Search PubMed
, article ID 178921.
- B. J. Privett, J. Youn, S. A. Hong, J. Lee, J. Han, J. H. Shin and M. H. Schoenfisch, Antibacterial fluorinated silica colloid superhydrophobic surfaces, Langmuir, 2011, 27, 9597–601 CrossRef CAS
.
- C. R. Crick, S. Ismail, J. Pratten and I. P. Parkin, An investigation into bacterial attachment to an elastomeric superhydrophobic surface prepared via aerosol assisted deposition, Thin Solid Films, 2011, 519, 3722–3727 CrossRef CAS
.
- L. R. Freschauf, J. McLane, H. Sharma and M. Khine, Shrink-induced superhydrophobic and antibacterial surfaces in consumer plastics, PLoS One, 2012, 7, e40987 CAS
.
- E. Fadeeva, V. K. Truong, M. Stiesch, B. N. Chichkov, R. J. Crawford, J. Wang and E. P. Ivanova, Bacterial retention on superhydrophobic titanium surfaces fabricated by femtosecond laser ablation, Langmuir, 2011, 27, 3012–3019 CrossRef CAS
.
- C. Sousa, D. Rodrigues, R. Oliveira, W. Song, J. F. Mano and J. Azeredo, Superhydrophobic poly(L-lactic acid) surface as potential bacterial colonization substrate, AMB Express, 2011, 1, 34 CrossRef CAS
.
- I. Banerjee, R. C. Pangule and R. S. Kane, Anti-fouling coatings: recent developments in the design of surfaces that prevent fouling by proteins, bacteria, and marine organisms, Adv. Mater., 2011, 23, 690–718 CrossRef CAS
.
- C. P. Stallard, K. A. McDonnell, O. D. Onayemi, J. P. O'Gara and D. P. Dowling, Evaluation of protein adsorption on atmosphere plasma deposited coatings exhibiting superhydrophilic to superhydrophobic properties, Biointerphases, 2012, 7, 31 CrossRef CAS
.
- P. Roach, N. J. Shirtcliffe, D. Farrar and C. C. Perry, Quantification of surface bound proteins by fluorometric assay: Comparison with quartz crystal microbalance and amido black assay, J. Phys. Chem. B, 2006, 110, 20572–20579 CrossRef CAS
.
- Y. Koc, A. J. Mello, G. McHale, M. I. Newton, P. Roach and N. J. Shirtcliff, Nano-scale superhydrophobicity: suppression of protein adsorption and promotion of flow-induced detachmeng, Lab Chip, 2008, 8, 582–586 RSC
.
- R. B. Pernites, C. M. Santos, M. Maldonado, R. R. Ponnapati, D. F. Rodrigues and R. C. Advincula, Tunable protein and bacterial cell adsorption on colloidally temperlated superhydrophobic polythiophene films, Chem. Mater., 2012, 24, 870–880 CrossRef CAS
.
- X. Wang, W. Song, Y. Gan, C. Cheng, C. Xia, P. Jiang, X. Deng, T. Sun and L. Jiang, Superhydrophobic modification of hydroxyapatitie decreased in vitro Streptococcus mutans adhesion and calcium dissolution, Chin. J. Dent. Res., 2009, 12, 15–20 CAS
.
- V. K. Truong, H. K. Webb, E. Fadeeva, B. N. Chichkov, A. H. F. Wu, R. Lamb, J. Y. Wang, R. J. Crawford and E. P. Ivanova, Air-directed attachment of coccoid bacteria to the surface of superhydrophobic
lotus-like titanium, Biofouling, 2012, 28, 539–550 CrossRef CAS
.
- J. Zhang, X. Sheng and L. Jiang, The dewetting properties of Lotus leaves, Langmuir, 2009, 25, 1371–1376 CrossRef CAS
.
- H. Zhang, R. Lamb and J. Lewis, Engineering nanoscale roughness on hydrophobic surface – preliminary assessment of fouling behaviour, Sci. Technol. Adv. Mater., 2005, 6, 236–239 CrossRef CAS
.
- J. Ma, Y. Sun, K. Gleichauf, J. Lou and Q. Li, Nanostructure on Taro leaves fouling by colloids and bacteria under submerged conditions, Langmuir, 2011, 27, 10035–10040 CrossRef CAS
.
- T. Liu, L. Dong, T. Liu and Y. Yin, Investigations on reducing microbiologically-influenced corrosion of aluminium by using superhydrophobic surfaces, Electrochim. Acta, 2010, 55, 5281–5285 CrossRef CAS
.
- P. V. Mahalakshmi, S. C. Vanithakumari, J. Gopal, U. K. Mudali and B. Raj, Enhancing corrosion and biofouling resistance through superhydrophobic surface modification, Current Science, 2011, 101, 1328–1336 CAS
.
- M. S. Khalil-Abad and M. E. Yazdanshenas, Superhydrophobic anti-bacterial cotton textiles, J. Colloid Interface Sci., 2010, 351, 293–298 CrossRef
.
- J. S. Chung, B. G. Kim, S. Shim, S. E. Kim, E. H. Sohn, J. Yoon and J. C. Lee, Silver-perfluorodecanethiolate complexes having superhydrophobic, antifouling, antibacterial properties, J. Colloid Interface Sci., 2012, 366, 64–69 CrossRef CAS
.
- H. Tang, H. Wang and J. He, Superhydrophobic titania membranes of different adhesive forces fabricated by electrospinning, J. Phys. Chem. C, 2009, 113, 14220–14224 CAS
.
- J. Zheng, J. Song, Q. Jiang and J. Lian, Superhydrophobic behaviour and optical properties of ZnO film fabricated by hydrothermal method, J. Mater. Sci. Technol., 2012, 28, 103–108 CAS
.
|
This journal is © The Royal Society of Chemistry 2013 |
Click here to see how this site uses Cookies. View our privacy policy here.