DOI:
10.1039/C3AN01632C
(Tutorial Review)
Analyst, 2014,
139, 32-43
Redox-capacitor to connect electrochemistry to redox-biology
Received
29th August 2013
, Accepted 20th October 2013
First published on 22nd October 2013
Abstract
It is well-established that redox-reactions are integral to biology for energy harvesting (oxidative phosphorylation), immune defense (oxidative burst) and drug metabolism (phase I reactions), yet there is emerging evidence that redox may play broader roles in biology (e.g., redox signaling). A critical challenge is the need for tools that can probe biologically-relevant redox interactions simply, rapidly and without the need for a comprehensive suite of analytical methods. We propose that electrochemistry may provide such a tool. In this tutorial review, we describe recent studies with a redox-capacitor film that can serve as a bio-electrode interface that can accept, store and donate electrons from mediators commonly used in electrochemistry and also in biology. Specifically, we (i) describe the fabrication of this redox-capacitor from catechols and the polysaccharide chitosan, (ii) discuss the mechanistic basis for electron exchange, (iii) illustrate the properties of this redox-capacitor and its capabilities for promoting redox-communication between biology and electrodes, and (iv) suggest the potential for enlisting signal processing strategies to “extract” redox information. We believe these initial studies indicate broad possibilities for enlisting electrochemistry and signal processing to acquire “systems level” redox information from biology.
Introduction
Biology routinely uses redox active compounds and redox reactions to perform critical functions; to harvest energy via oxidative phosphorylation, to defend against pathogenic threats via an oxidative burst, to metabolize toxins via phase I reactions, to generate diffusible and membrane-permeable signaling molecules (i.e., NO and H2O21,2), and to switch protein conformations or modify protein–protein interaction through thiol–disulfide reactions.3–5 In some cases, the importance of redox reactions in biology is well-established but in other cases the roles/mechanisms of redox reactions are still emerging6,7 and putative biological activities are sometimes controversial.8–11 A key challenge in redox biology is that many important redox reactions remain obscured from measurement.
Characterizing the “flow” of electrons in biology is more challenging than measuring currents in an electric circuit because free electrons don't generally exist in aqueous solutions: rather electrons must be transferred via chemical species through oxidation–reduction reactions. A traditional approach to redox biology is to measure individual chemical species (reactants, intermediates and products), and examine the redox-state of important redox-couples (e.g., NADP/NADPH). However, many of these chemical species are only marginally stable and have differing lifetimes, and thus a suite of analytical methods is often required. This point is illustrated by reactive oxygen species (ROS) where the one electron reduction of O2 yields superoxide (O2˙−), a two electron reduction yields hydrogen peroxide (H2O2), and interactions between H2O2 and metals yields the hydroxyl radical (OH˙).12 Characterizing the mechanisms of ROS generation and the myriad of resulting reactions/activities poses a formidable analytical challenge.13,14
We believe electrochemistry provides an intriguing set of capabilities that could potentially be applied for analyses in redox biology.15–18 The range of capabilities that electrochemical methods offer are listed in Table 1 with simplicity, speed and sensitivity being well-recognized attributes of electrochemical analyses. To suggest the potential of electrochemistry, we consider an analogy with the historical example from neurobiology. Over 60 years ago, microelectrodes enabled researchers to observe that neuronal cells signal through millisecond bursts of ionic currents across nm distances (i.e., across the cell membrane).19 These measurements were made before gene cloning, before the DNA's duplex structure was established and before the chemiosmotic theory was accepted. Even with today's advanced instrumentation, it is difficult to imagine how action potentials could be measured over such time and length scales without the use of microelectrodes.
Table 1 Attractive features of electrochemical analyses
• The instrumentation is relatively inexpensive, robust and potentially portable |
• The time scales that can be probed extend over many orders of magnitude (including biologically-relevant time scales) |
• The voltage imposed at an electrode can be precisely set to promote the transfer of electrons across the electrode-solution interface to oxidize or reduce key redox species (i.e., to switch their redox state) |
• The imposed electrode voltage can be held constant, rapidly switched, oscillated or programmed to provide an arbitrarily complex sequence of input redox signals |
• The measured output currents provide a very sensitive quantification of the rate of redox reactions |
• The input–output is in a convenient form for signal processing analyses |
Electrode systems provided transformative capabilities for neurobiology and medicine (e.g., electrocardiograms, ECG) by providing a simple, sensitive and spatiotemporally-selective means to measure electrical signatures associated with ionic currents. In our research, we ask: is it possible to apply electrode systems to observe biologically-relevant signatures associated with the flow of electrons? The conventional concern with electrochemical measurements is their lack of selectivity. When complex matrices (e.g., blood) are measured, it is generally not possible to relate the measured current at the electrode to a single redox-active component, nor to partition the observed currents into the individual contributions of each component in the mix. Yet, this lack of selectivity does not preclude the extensive use of (micro)electrodes to measure ionic currents – the observed electrical signatures of the ECG provide useful information even without knowing the individual contributions from the Cl−, Na+ or Ca2+ ions.
Our broad hypothesis is that electrochemistry may provide a simple, sensitive and dynamic means to obtain systems-level redox information. We anticipate that such information could complement detailed chemical information obtained from traditional instrument-intensive approaches. Further, we suggest the possibility that electrochemical measurements that access biologically-relevant redox information may provide useful, systems-level, information of redox-state and/or redox context. We believe our contribution to this pursuit is the use of a bio-based redox-capacitor that facilitates redox-communication across the biology–electrode interface. The fabrication and capabilities of this catechol-based redox-capacitor is the focus of this tutorial review.
Catechols
Redox-active components for biology and technology
Catechols and phenols are among the most abundant organics in nature20 and are emerging as important materials for technology.21–27 In many cases, natural catechols/polyphenols are known to possess redox activities although a comprehensive understanding of these redox activities and their biological relevance has been elusive.28–30 Currently, the redox (i.e., antioxidant) activities of dietary phenols31,32 attract the most attention, however it is interesting to note that forty years ago, melanins were reported to be amorphous semiconductors33,34 and more recently, lignin derivatives were reported to improve the performance of conducting polymers.35 The important point is that phenols and especially catechols readily accept, store and donate electrons under biologically-relevant conditions and these redox-activities may enable an “electronic” interfacing of biology to an electrode. Several years ago, we investigated a biomimetic approach to generate catechol-matrices36,37 and more recently we observed that these matrices possess redox-activity.38
Fabrication of catechol–chitosan redox-capacitor
Catechols and the aminopolysaccharide chitosan are used to fabricate our redox-capacitor. Scheme 1 indicates that catechols are readily oxidized to o-quinones which are reactive electrophiles. In our studies, we generate these quinones electrochemically or enzymatically and then allow them to react with the nucleophilic amines of the aminopolysaccharide chitosan. Chitosan is a particularly versatile material because it possesses a unique combination of properties.39 In addition to conferring nucleophilic properties, chitosan's amines confer pH-responsive properties to this polysaccharide: at low pH when the amines are protonated chitosan is water soluble but at higher pH's the amines become deprotonated and chitosan can form a three-dimensional hydrogel network. Chitosan's pH-responsive, film-forming properties allow it to be easily cast or electrodeposited into films,40–46 while chitosan's nucleophilic properties permit o-quinone grafting.47–52
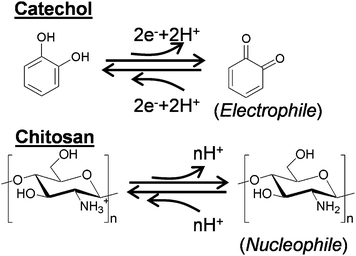 |
| Scheme 1 Materials used to fabricate the redox-capacitor. Catechols can be readily (and reversibly) oxidized, and the oxidized quinone can be covalently grafted to chitosan. Chitosan's pH-responsive film forming properties enable its electrodeposition, while its nucleophilic properties permit quinone grafting. | |
Scheme 2a illustrates an electrochemical method for grafting catechols to chitosan. A chitosan hydrogel film is first cast or electrodeposited onto an electrode and this chitosan-coated electrode is immersed in a solution containing a catechol. The catechol can diffuse through the chitosan hydrogel and be oxidized at the underlying electrode if the voltage is greater than +0.3 V vs. Ag/AgCl, and then the quinone product can diffuse from the electrode and react with chitosan's amines.36,37Scheme 2b illustrates an analogous enzymatic approach for generating catechol–chitosan films. In this case, the enzyme tyrosinase is able to convert phenols with a single aromatic hydroxyl or catechols into o-quinones that can diffuse into the chitosan film to undergo reaction.53 Quinone grafting to chitosan is complex and likely involves Michael-type adduct and Schiff base chemistries as suggested in Scheme 2c.54–56
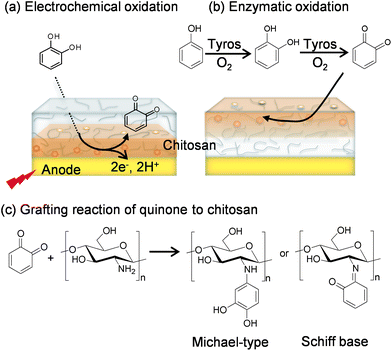 |
| Scheme 2 Fabrication of catechol–chitosan films. A catechol is (a) electrochemically oxidized or (b) enzymatically oxidized by tyrosinase (Tyros), and the diffusible oxidation product (i.e., o-quinone) grafts to the nucleophilic amines of the aminopolysaccharide chitosan. (c) Quinone grafting likely involves Schiff base and Michael-type adduct formation although a complex array of grafted moieties may be generated. | |
Properties conferred by redox-active catechol-chitosan matrices
Initial studies demonstrated that the catechol–chitosan films are non-conducting as they cannot exchange electrons directly with the underlying electrode.38 Presumably, these films are electronically non-conducting because the grafted catechols are neither “connected” to each other to form an extended conjugated structure nor “connected” to the electrode surface in these ≈1 μm thick films (≈0.3 μm dry thickness). The lack of electronic conductivity for the catechol–chitosan films appears to contrast with behaviors observed for more familiar redox-polymers where electrons can be “self-exchanged” among the polymer-based redox centers.57–59
Although electronically non-conducting, the catechol-modified chitosan films are hydrogels that allow the diffusion of ions and small molecules (i.e., the films are ionically conducting). As indicated in Scheme 3, these films are also redox-active. In particular, the films can be switched between oxidized (Q) and reduced (QH2) states by exchanging electrons with diffusible mediators in a thermodynamically-constrained process (i.e., electrons must flow from more negative to more positive potentials). As indicated in Scheme 3, the Q state is presumably the o-quinone form of the grafted moieties while the QH2 state is presumably the catechol form (we note that a complex array of moieties may be grafted to the films). Consistent with this view is that the redox potential of the grafted moieties has been estimated to be +0.2 V vs. Ag/AgCl38 which is in agreement with the redox potential of typical catechol/quinone redox couples.60
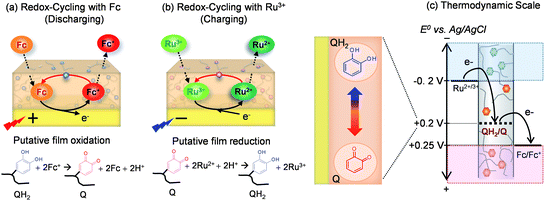 |
| Scheme 3 Redox-active catechol–chitosan films can exchange electrons with diffusible mediators in thermodynamically-constrained redox-reactions. (a) Redox-cycling with ferrocene dimethanol (Fc) can discharge the catechol–chitosan film. (b) Redox-cycling with Ru(NH3)6Cl3 (Ru3+) can charge the film. (c) Thermodynamic scale shows electrons “flow” from more-negative to more-positive redox-potentials. | |
Scheme 3a shows that the common electrochemical mediator, ferrocene dimethanol (designated Fc), can diffuse through the chitosan film, donate an electron to the electrode at potentials above about +0.25 V (vs. Ag/AgCl). The oxidized Fc+ can diffuse out of the film into the bulk solution or undergo a redox-cycling reaction within the film by accepting electrons from the grafted QH2 moieties. This Fc-mediated redox-cycling reaction serves to “discharge” electrons from the film by converting QH2 to Q moieties. Scheme 3b shows an analogous redox-cycling mechanism with the electrochemical mediator Ru(NH3)6Cl3 (designated Ru3+) which can be reduced by the electrode and then transfer these electrons to the film in a “charging” reaction that converts Q to QH2 moieties.
Scheme 3c shows the relevant thermodynamic scale for these various electron transfer steps. As indicated in Scheme 3, thermodynamics requires electrons to “flow” from more negative to more positive redox potentials. Also indicated in Scheme 3 is that the potential (i.e., voltage) of the electrode can be set independently to control the net direction of electron flow (either to the electrode or from the electrode).
Thus, Scheme 3 illustrates that the catechol–chitosan film can store electrons (as QH2 moieties) and these electrons can be transferred from the film (Scheme 3a) or transferred to the film (Scheme 3b) through independent mediator-based redox-cycling reactions. There are four important properties that result from these redox-cycling reactions.
Amplification of mediator currents
The catechol–chitosan film can amplify mediator-based output currents. Amplification is illustrated in Fig. 1a which shows cyclic voltammograms (CVs) for catechol–chitosan-coated gold electrodes incubated in solutions containing both the Fc and Ru3+ mediators. Peak output currents are observed in both the regions of Fc oxidation and Ru3+ reduction. Amplification is apparent by comparing this CV with those from two controls, a bare gold electrode and an electrode coated with a chitosan film (without catechol grafting), each in solutions containing the Fc plus Ru3+ mediators. Again, peak currents are observed in the region associated with Fc oxidation and Ru3+ reduction, however the peak currents are considerably less relative to those observed for the catechol–chitosan film. A final control for a catechol–chitosan film incubated without mediators shows no discernible peak currents which is consistent with the explanation that catechol–chitosan films are non-conducting (i.e., mediators are required for electron exchange with the film).
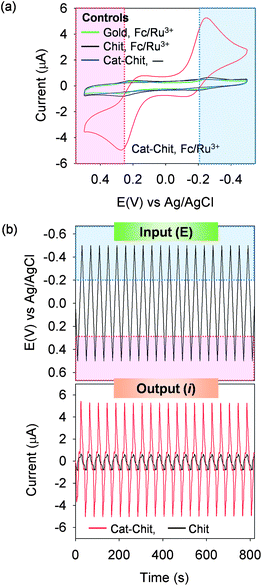 |
| Fig. 1 The redox-cycling reactions in the catechol–chitosan film amplify mediator currents. (a) Cyclic voltammograms for catechol–chitosan films incubated with both Fc and Ru3+ mediators show amplification compared to controls. (b) Input–output representation for multiple CV-cycles. | |
Fig. 1b plots an analogous sequence of multiple CVs as input–output curves in which time is explicitly shown. Irrelevant to how the data is represented, the results demonstrate amplified output currents for the catechol–chitosan film incubated with both mediators. This amplification is a direct result of the redox-cycling reactions of Scheme 3.
Partial-rectification of mediator currents
A second property of the catechol–chitosan film is that it can partially-rectify mediator currents. Partial rectification is illustrated in Fig. 2a which shows CVs for catechol–chitosan films incubated in solutions containing only a single mediator, Fc. In this case, the films display significant oxidation peaks as the Fc redox-cycling reaction of Scheme 3a serves to discharge the films. In the absence of a reducing mediator, the film cannot be re-charged and small reducing currents are observed (compared to the oxidation currents). Also observed in Fig. 2a, is that the oxidizing current progressively decreases over time as the film becomes progressively discharged. This decay in oxidation currents is more obvious in the input–output plots in Fig. 2b.
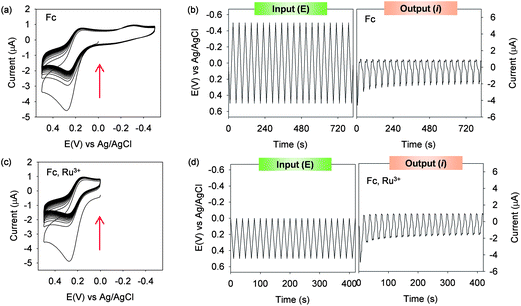 |
| Fig. 2 Partial rectification of mediator currents. (a) Cyclic voltammograms and (b) input–output curves for catechol–chitosan films incubated with the Fc but not the Ru3+ mediator: these conditions allow the films to be discharged but not charged. (c) Cyclic voltammograms and (d) input–output curves for catechol–chitosan films incubated with both Fc and Ru3+ mediators but with an input potential range that is unable to reduce Ru3+: these conditions also allow film discharging but not charging. | |
In an analogous experiment catechol–chitosan films were incubated with both the Fc and Ru3+ mediators but the input potential cycle was set to remain more positive than the E° for the Ru3+ mediator. Under these conditions, the Ru3+ mediator could not be reduced and the redox-cycling reaction in Scheme 3b could not be engaged. Fig. 2c and d show that an Fc oxidation peak is observed, a minimal reduction peak is observed, and there is a progressive decrease in the Fc oxidation peak as the film becomes progressively discharged.
In summary, the experiments outlined in Fig. 2 illustrate that by selectively engaging the redox-cycling reactions of Scheme 3 it is possible to obtain significant currents in one direction (e.g., oxidation) while suppressing currents in the opposite direction. This partial rectification is not an intrinsic feature of the films but depends on film's redox-context (state); whether the film has electrons to donate for discharging (QH2 → Q), or has oxidized sites to accept electrons for charging (Q → QH2).
Gated output currents
Because the catechol–chitosan film is electronically non-conducting, a mediator is required to charge the film (e.g., Ru3+) and a separate mediator is required to discharge the film (e.g., Fc). As a result, the properties of the mediators (i.e., the mediators' E°) control charging and discharging. This behavior is illustrated in Fig. 3a which shows CVs for catechol–chitosan films incubated with either Ru3+ (charging) plus Fc (discharging), or Ru3+ (charging) plus acetosyringone (AS; discharging). When Fc (E° ≈ +0.25 V) was used, the oxidative discharging current is drawn when the potential exceeds about +0.1 V. In contrast, when AS (E° ≈ +0.5 V) was used, the discharging current is not drawn until the potential exceeds about +0.4 V. This difference in discharging potential results because the mediator must be oxidized to initiate film discharging (by the redox-cycling mechanism in Scheme 3a) and a larger potential is required to oxidize AS relative to Fc. Thus, Fig. 3a shows that the oxidative mediator (Fc or AS) “gates” the output and this gating is controlled by the mediator's E°. Fig. 3b shows that this gating also significantly alters the shape of the output curve.
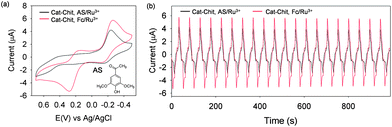 |
| Fig. 3 Mediator gating of the output currents. (a) CVs for catechol–chitosan films incubated with two mediators (either Fc plus Ru3+, or AS plus Ru3+): because AS has a higher E° than Fc (+0.5 vs. +0.25 V) a higher electrode potential is required to discharge the catechol–chitosan film. (b) Input–output curves are significantly altered depending on the mediators. | |
Steady input–output curves
Fig. 1b shows that reproducible output pattern is generated under conditions in which both redox-cycling mechanisms are engaged by the catechol–chitosan film. Under these conditions, the film is sequentially charged and discharged in each cycle. Under conditions in which charging and discharging are not balanced (e.g., when only Fc is used as in Fig. 2b), the shape of the output curve is not steady but changes over time. Non-steady output is more readily identified by integrating the current with respect to time and displaying the charge transfer (Q = ∫idt). Fig. 4a shows the input potential and output charge transfer for the catechol–chitosan films from Fig. 1b (steady output) and Fig. 2b (non-steady output). Fig. 4b shows the non-steady output for experiments from Fig. 2d in which the potential input did not allow Ru3+ redox-cycling to charge the film.
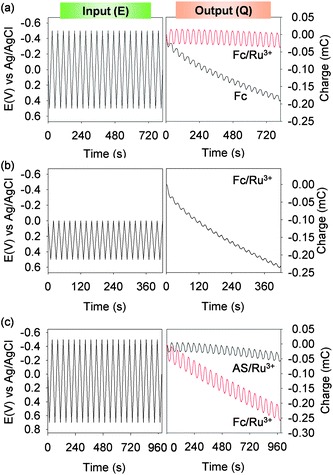 |
| Fig. 4 “Steady” (i.e., time independent) output curves can be generated by balancing interactions among the input potential, the mediators, and the catechol–chitosan film. (a) Charge transfer curves plotted for the results in Fig. 1b and 2b. (b) Non-steady charge transfer for the results in Fig. 2d. (c) Charge transfer for the results in Fig. 3 illustrate the importance of input potential and mediator interactions on output. | |
Fig. 4c shows the charge transfer for the results in Fig. 3. In this case, the net charge transfer with acetosyringone (AS) and Ru3+ is nearly zero while the net charge transfer with Fc and Ru3+ shows a net oxidation current occurs. This net oxidation with Fc and Ru3+ occurs for the experiment in Fig. 4c (and not Fig. 4a) because the potential range in the experiment of Fig. 4c was extended to a larger oxidative potential (vs. conditions in Fig. 4a). While this extended potential range in Fig. 4c allows a comparison of the behavior of AS (more difficult to oxidize; higher E°) and Fc (more easily oxidized; lower E°) it also exposes the Fc-containing solution to greater oxidative conditions (compared to conditions in Fig. 4a) and results in a non-steady output charge transfer.
The results in Fig. 4 illustrate that interactions among the film, mediators and applied potential control the output. Further, these results demonstrate the opportunity to impose cyclic inputs to generate steady outputs – a common signal processing approach to acquire information.
Catechol–chitosan films behave as redox-capacitors
In summary, the above text describes how electrochemical mediators can interact with a redox-active catechol–chitosan film to engage independent redox-cycling capabilities. In some cases, the redox-cycling serves to donate electrons to the film where these electrons can be stored as a reduced (QH2) state of the film. In other cases, the redox-cycling serves to accept electrons and discharge the film. The ability of the film to accept, store and donate electrons is characteristic of a redox-capacitor. Thus, catechol-modification of chitosan films provides a simple means to generate a redox-capacitor. Importantly, interactions between the mediators and this capacitor-film dramatically alter output currents in ways that are readily explained by the simple thermodynamically-driven redox-cycling reactions outlined in Scheme 3. In the next section we describe the relevance of this redox-capacitor to biology.
Connecting to biologically relevant redox-reactions
Somewhat surprisingly, we observed that the catechol-modified chitosan films could exchange electrons with biologically-relevant oxidants and reductants.61 Potentially, the abilities of the catechol–chitosan films to interact with both electrochemical mediators and biological electron donors/acceptors will provide interesting opportunities to bridge redox-communication between biology and electronics.
Enzymatic charging
Fig. 5a illustrates that we initially tested two common biological reductants; ascorbate (vitamin C) which can be found extracellularly (e.g., in the plant apoplast62 or animal bloodstream63), and NADPH which is a common intracellular reducing agent. In both cases, these reductants were observed to donate their electrons to charge the catechol-modified film.61 The ability of the film to exchange electrons with NADPH suggested the potential to charge the film through an enzyme-catalyzed redox-cycling scheme illustrated in Fig. 5b. In this case, glucose dehydrogenase (GDH) catalyzes the transfer of electrons from glucose to NADP+ to generate NADPH, the reduced NADPH then diffuses into the film where it donates its electrons to re-generate the oxidized NADP+ form. To quantify the transfer of electrons to the film, we employed an electrochemical “titration” method involving the Fc mediator as suggested in Fig. 5b. Fig. 5c shows experimental results in which the amount of electrons donated into the film (NFilm,Charged) varied depending on the glucose content of the solution (further details of the chronocoulometric measurement are described in the original publication).64 Thus, the catechol–chitosan matrix can be charged both electrochemically or biologically (i.e., enzymatically).
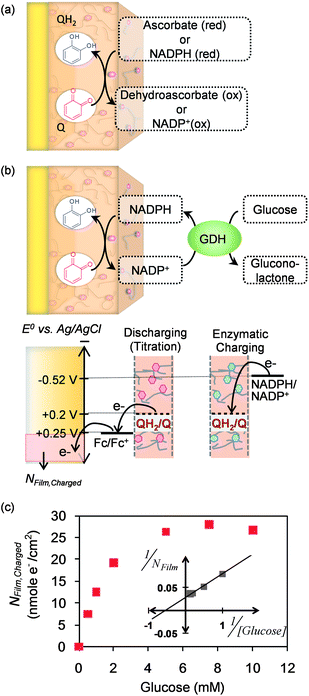 |
| Fig. 5 Biological reductants can donate their electrons to charge the catechol–chitosan film. (a) Schematic illustrating film charging by ascorbate (extracellular reductant) and NADPH (intracellular reductant). (b) Schematic of enzymatic charging of the film by the transfer of electrons from glucose to the film through an NADPH redox-cycling mechanism. The thermodynamic plot illustrates the basis for the electrochemical titration that quantifies the number of electrons transferred to the film (NFilm,Charged). (c) Experimental results demonstrate enzymatic charging as a function of glucose concentration. Reprinted with permission from ref. 64. Copyright (2012) Wiley-VCH. | |
Oxidative discharging
Molecular oxygen is the common biological oxidant, and initial studies demonstrated that the catechol–chitosan film can donate electrons to partially reduce O2 to generate reactive oxygen species (ROS) as illustrated in Fig. 6a.61 Experimentally, we measured H2O2-generation and evaluated films that had been fabricated to have differing levels of grafted catechol moieties (NCatechol; details of film fabrication and characterization are described in the original publication).61 The first observation in Fig. 6b is that films prepared without catechol (i.e., NCatechol = 0; control chitosan films) were unable to generate H2O2. This observation is expected because chitosan is redox-inactive and thus cannot accept and store electrons nor can it donate electrons to O2. The second observation is that films fabricated with more grafted catechols could generate more H2O2 (upper curve in Fig. 6b). Finally, the film's ability to generate H2O2 depended on its redox state; films that had been initially prepared in a reduced (QH2) state were poised to donate these electrons to O2, while films that were prepared in an oxidized (Q) state had no electrons to donate to O2 and thus generated no H2O2. [Note: the films' redox state was set electrochemically prior to exposing them to O2 as described in the original publication.61]
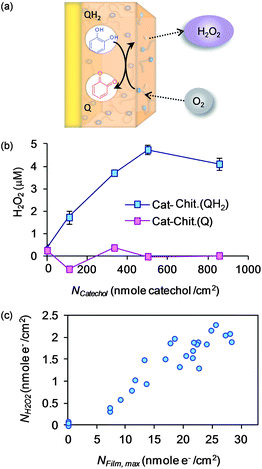 |
| Fig. 6 The catechol–chitosan film can be discharged by donating its electrons to O2 to generate reactive oxygen species (i.e., H2O2). (a) Schematic illustrating film discharging by O2. (b) Experimental results demonstrating that catechol grafting confers the H2O2-generating ability of the film, and that H2O2-generation requires that the film was poised in a reduced (QH2) state (i.e., the Q-state has no electrons to donate). (c) Correlation between H2O2-generation and the film's redox-capacity (NFilm,max is an experimental measure of the number of electrons that can be stored by the film). Reprinted with permission from ref. 61. Copyright (2011) American Chemical Society. | |
The correlation in Fig. 6c further emphasizes the relationship between the film's ability to store electrons (NFilm,max; redox capacity as measured electrochemically) and the film's ability to donate electrons to generate H2O2 (NH2O2). In summary, the results in Fig. 6 indicate that the catechol–chitosan films can donate electrons to O2 and generate a reactive oxygen species (H2O2) that is widely believed to be integral to biological signaling1,2 and effector actions.12
Opportunities for analysis
Individual analyses of chemical species (or classes)
The generation of reactive oxygen species for defense (e.g., the oxidative burst immune responses) and signaling are well-recognized, yet biological systems produce a range of additional redox-active metabolites that are receiving increasing attention. Phenazines are one the most well-studied classes of redox-active bacterial metabolites65,66 and these metabolites are believed to; (i) allow the producing Pseudomonas to mediate signaling among cells (i.e., quorum sensing67), (ii) transfer electrons outside the cell for redox-balancing,68 and (iii) maintain redox-homeostasis of multicellular biofilms.69 One such phenazine, pyocyanin (PYO), is also a virulence factor for the opportunistic pathogen P. aeruginosa. It is believed that this metabolite is secreted by the bacteria, accepts electrons from host cells and then transfers these electrons to O2 in a redox-cycling operation that both disrupts the host cell's redox-state and generates ROS.67,70,71 Importantly, P. aeruginosa is emerging as one of the most significant pathogens of nosocomial (hospital acquired) infections, especially for burn patients.72–74 A rapid detection of this pathogen could be integral to successfully identifying and treating infections in this vulnerable patient population.
Because of PYO's redox-activity, electrochemistry provides a simple, rapid and sensitive means to assay this metabolite75–78 and thus to detect the presence of the producing P. aeruginosa. Fig. 7a indicates that PYO is thermodynamically capable of donating electrons to catechol–chitosan film and could undergo redox-cycling in the film to amplify outputs and facilitate detection. [Redox-cycling is a common amplification approach to enhance the sensitivity of electrochemical detection.79,80] As suggested in Fig. 7a, a “steady” amplified output requires that the PYO-mediated redox-cycling that charges the film (during the reducing portion of the cycle) must be balanced by a redox-cycling mechanism that discharges the film (during the oxidative portion of the cycle.) In our studies, we used Fc because this mediator is reported to be compatible with biological systems.
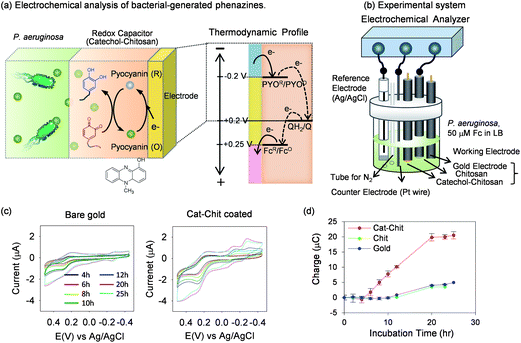 |
| Fig. 7 Electrochemical analysis of bacterial-generated phenazines (i.e., pyocyanin, PYO). (a) Thermodynamic scale indicating that PYO can transfer electrons to the catechol–chitosan film. (b) The experimental system that allows in situ electrochemical measurements during bacterial cultivation. (c) In situ cyclic voltammograms (CVs) at various times during P. aeruginosa cultivation show that catechol–modified film can amplify output (vs. control gold electrode). (d) Chronocoulometric measurements that compared the amplified output for the catechol–chitosan film to controls. Reprinted with permission from ref. 81. Copyright (2013) American Chemical Society. | |
To test the ability of the catechol–chitosan film to generate amplified outputs for PYO detection we used the experimental system shown in Fig. 7b. This system allows the bacteria to be cultured and electrochemical monitoring to be performed in situ. To facilitate comparison of the electrochemical measurements, we equipped this system with three different working electrodes; a bare gold electrode, a gold electrode coated with an unmodified chitosan film, and a gold electrode coated with a catechol–chitosan film. As shown in Fig. 7b, this system was also equipped with a reference electrode (Ag/AgCl) and counter electrode (Pt wire).
P. aeruginosa was inoculated into standard bacterial cultivation medium that was supplemented with 50 μM Fc. During cultivation, CV measurements were made intermittently using the three electrodes. Before each measurement, N2 was bubbled into the system to minimize interference from O2 which can be electrochemically reduced at the same potentials as PYO. Fig. 7c compares CVs for a bare gold electrode and the electrode coated with the catechol–chitosan film at seven different time points. These measurements indicate a substantial amplification for the electrode coated with the catechol–chitosan film consistent with a redox-cycling of PYO in this film.
While cyclic voltammetry is the most common electrochemical measurement, various other methods can be used to enhance sensitivity, facilitate quantification, or extract additional information. We performed in situ chronocoulometric measurements during the course of cultivation and compared the results for the three electrodes (further details are described in the original publication81). Fig. 7d shows that measured charge for the bare gold and the electrode coated with an unmodified chitosan film are both very small. As expected, the output charge is substantially amplified for measurements made with the electrode coated with the catechol–chitosan film.
In summary, the results in Fig. 7 demonstrate that the redox-active metabolite pyocyanin (PYO) can shuttle electrons from bacterial cells to the catechol–chitosan matrix where the electrons can be stored: thus, PYO provides a means for this living system to charge the catechol–chitosan redox-capacitor. By a judicious selection of a discharging mediator (e.g., Fc) and applied electrode potentials, it is possible to use this redox-capacitor to generate amplified outputs for the detection of PYO which might allow the early detection of infections by the opportunistic pathogen P. aeruginosa. In addition, the catechol–chitosan matrix is simple to fabricate and employs biocompatible materials and thus allows for in situ measurements which may be useful for studying the spatiotemporal dynamics of PYO generation in complex systems (e.g., bacterial biofilms).
Global analysis of redox context
The ease with which the catechol-modified chitosan interacts with redox-active molecules suggests to us that it offers a new opportunity to access redox information from biology and probe this information using signal processing strategies. Our initial effort is illustrated in Fig. 8a which suggests that cyclic potential inputs are transferred to a biological system through mediators while the outputs depend on redox-context.82 It is important to note that the application of cyclic inputs to generate “steady” cyclic outputs is a common feature of signal processing. The catechol–chitosan film serves to process this information (through its amplification, rectification and gating properties) in ways that facilitate interpretation. As an initial test of this concept, we used the experimental system of Fig. 8b and provided differing biological contexts (either with or without a population of E. coli) and differing oxidative contexts (aerobic or anaerobic). Fig. 8c indicates that the system was augmented with two biological mediators; the bacterial phenazine pyocyanin (for film-charging), and the plant phenol acetosyringone (AS; for film discharging). Also indicated in Fig. 8c is that a cyclic potential was imposed by the electrode to sequentially charge and discharge the film.
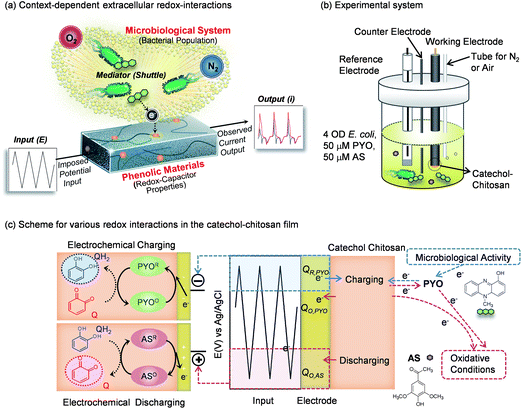 |
| Fig. 8 Global analyses of redox-context. (a) Schematic illustrating that cyclic electrical inputs–outputs are transferred via mediators to the aqueous/biological system, while the catechol–chitosan interface “processes” this information. (b) Experimental system in which redox-context is varied. (c) Schematic illustrating the various redox interactions in the catechol–chitosan film and the solution. Adapted with permission from ref. 82. Copyright (2013) American Chemical Society. | |
Cyclic voltammograms (CVs) and input–output curves for these four conditions are shown in Fig. 9a.82 These plots demonstrate that the observed output is substantially different depending on the redox-context being probed. As an initial effort to correlate the data we examined three regions of the CVs as illustrated in Fig. 9b: the regions where PYO is electrochemically reduced and electrochemically oxidized, and the region where AS is electrochemically oxidized (little AS reduction is apparent from the CVs). The currents in these regions were integrated with respect to time to determine the charge transfer (Q = ∫idt) in these three regions. These values were then combined to give either a rectification ratio for pyocyanin (RRPYO = QR,PYO/QO,PYO) or the fraction of electrochemical oxidation occurring in the pyocyanin region (FPYO). The following discussion provides a rationale for these correlating parameters while the interested reader is referred to the original publication for a more extended discussion.82
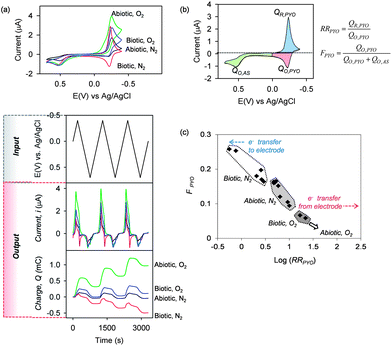 |
| Fig. 9 Experimental results and correlation of global redox context. (a) CVs and input–output curves for four experimental contexts depending on the presence/absence of O2 (aerobic/anaerobic) and presence/absence of E. coli (biotic/abiotic). (b) Parameters calculated from the CVs that correlate data based on rectification of pyocyanin currents and the fraction of total electrochemical oxidation that is attributed to pyocyanin. (c) Observed correlation for the four experimental contexts. Reprinted with permission from ref. 82. Copyright (2013) American Chemical Society. | |
Fig. 9c shows the correlation generated using these four experimental conditions. Under abiotic-anaerobic conditions (Abiotic, N2), the RRPYO > 1 (i.e., log[RRPYO] > 0) because pyocyanin is reduced exclusively at the electrode but pyocyanin is re-oxidized both by transferring electrons to the film and back to the electrode (thus, QO,PYO < QR,PYO). When E. coli is added under these anaerobic conditions, then pyocyanin is reduced both by the bacteria and by the electrode (i.e., the numerator in RRPYO is diminished). In addition, high metabolic activity under these Biotic, N2 conditions can generate excess reduced pyocyanin that is re-oxidized at the electrode (i.e., the denominator in RRPYO is increased). Thus, under these Biotic, N2 conditions the RRPYO is diminished and can even become less than one (i.e., log[RRPYO] can become negative). Under such Biotic, N2 conditions, the fraction of oxidation occurring in the pyocyanin region also increases and thus the data clusters in the upper left region of Fig. 9c for these Biotic, N2 conditions.
Aerobic conditions substantially alter the redox measurements since O2 can accept electrons from the bacterial culture (if present), from the catechol–chitosan film (e.g., Fig. 6a) and from pyocyanin itself. Under Abiotic, O2 conditions, the electrochemically-reduced pyocyanin can donate its electrons to both the film and to O2, and thus the electrochemical re-oxidation of pyocyanin is suppressed (QO,PYO → 0) and cannot be accurately calculated as suggested by the arrow at the lower right in Fig. 9c. Finally, under Biotic, O2 conditions, the results cluster in an intermediate region in Fig. 9c.
In summary, the experiments in Fig. 9 were set to provide differing redox contexts while the oscillating inputs were used to generate outputs that could be analyzed.82 The correlation observed in Fig. 9c is encouraging and suggests that information of the redox-context could be extracted from the output curve. Currently, we are examining more complex analysis in the hope that richer information can be extracted from these signals. Ultimately, our hope is that this analysis will provide a new approach to extract information from systems that are not well understood and are difficult to probe by conventional methods (e.g., the microbiome83).
Conclusions and future prospects
Electrochemistry enables chemical information to be acquired simply, rapidly and with high sensitivity. Further, the information acquired by electrochemistry is in a convenient form for analysis through high-powered signal processing methodologies. Catechol–chitosan films assembled at an electrode surface provide a unique combination of properties (switching, amplification, gating and rectification) that serve to “process” the electrochemical information. Because these films can exchange electrons with biologically-relevant oxidants and reductants, we anticipate that the information-processing capabilities of the catechol–chitosan-coated electrodes can be applied to studies in redox-biology: a field in need of simple analytical methodologies. Importantly, the catechol–chitosan films are easily assembled at the electrode interface because chitosan can be electrodeposited and catechols can be electrochemically-grafted (i.e., electrode-imposed signals can be used to fabricate the films at the electrode interface). In addition, the mediators (i.e., shuttles) that enable redox-communication with the film can be derived from biology and in some cases may be suitable for in situ analyses (e.g., the redox-active metabolites pyocyanin and acetosyringone were used in the experiments of Fig. 9).
In summary, we envision an electrochemical tool that provides access to global information of redox-conditions. To illustrate this vision, we close with an imperfect analogy – the electrochemical measurement of pH which is a global measure of acidity/basicity. The measured pH (along with information on pKa) provides insights of the speciation of molecules that have weak acidic and basic moieties (e.g., the net charge of a protein can be estimated). Thus, a pH measurement allows the charged state of a molecule in solution to be estimated and enables reliable prediction of how this molecule will undergo electrostatic interactions in its local environment. Further, pH measurements are often used as a qualitative (or semi-quantitative) measure of metabolic activity (e.g., acid build-up). We envision the catechol–chitosan film could be useful since it facilitates electron exchange with a broad range of oxidants and reductants and thus may provide a means to globally sample redox-information just as a pH electrode globally samples acid-base information. Potentially such a global measure of redox could provide insights on; (i) the oxidative/reductive stress being exerted on a biological system (e.g., during tumor therapy), (ii) the oxidative/reductive actions being taken by a biological system (e.g., oxidative burst or redox signaling), (iii) the driving force for biologically-relevant events (e.g., for disulfide bond formation/cleavage), and (iv) the redox interactions among chemical components (e.g., between drugs and antioxidants in our diet). Obviously much is needed to test the validity and utility of such a global redox measurement for biology.
Acknowledgements
The authors gratefully acknowledge financial support from the Robert W. Deutsch Foundation and the US Department of Defense (DTRA BO085PO008 and ONR N000141010446).
References
- E. Santiago, C. Contreras, A. García-Sacristán, A. Sánchez, L. Rivera, B. Climent and D. Prieto, Free Radical Biol. Med., 2013, 60, 136–146 CrossRef CAS PubMed.
- T. Finkel, J. Biol. Chem., 2012, 287, 4434–4440 CrossRef CAS PubMed.
- A. Bindoli and M. P. Rigobello, Antioxid. Redox Signaling, 2013, 18, 1557–1593 CrossRef CAS PubMed.
- P. Nagy, Antioxid. Redox Signaling, 2013, 18, 1623–1641 CrossRef CAS PubMed.
- T. Finkel, J. Cell Biol., 2011, 194, 7–15 CrossRef CAS PubMed.
- C. S. Pillay, J. H. Hofmeyr, L. N. Mashamaite and J. M. Rohwer, Antioxid. Redox Signaling, 2013, 18, 2075–2086 CrossRef CAS PubMed.
- A. R. Cyr and F. E. Domann, Antioxid. Redox Signaling, 2011, 15, 551–589 CrossRef CAS PubMed.
- M. Levine, M. G. Espey and Q. Chen, Free Radical Biol. Med., 2009, 47, 27–29 CrossRef CAS PubMed.
- M. A. Kohanski, D. J. Dwyer, B. Hayete, C. A. Lawrence and J. J. Collins, Cell, 2007, 130, 797–810 CrossRef CAS PubMed.
- I. Keren, Y. Wu, J. Inocencio, L. R. Mulcahy and K. Lewis, Science, 2013, 339, 1213–1216 CrossRef CAS PubMed.
- Y. Y. Liu and J. A. Imlay, Science, 2013, 339, 1210–1213 CrossRef CAS PubMed.
- J. A. Imlay, Nat. Rev. Microbiol., 2013, 11, 443–454 CrossRef CAS PubMed.
- C. Nathan and A. Cunningham-Bussel, Nat. Rev. Immunol., 2013, 13, 349–361 CrossRef CAS PubMed.
- B. C. Dickinson and C. J. Chang, Nat. Chem. Biol., 2011, 7, 504–511 CrossRef CAS PubMed.
- M. Ganesana, J. S. Erlichman and S. Andreescu, Free Radical Biol. Med., 2012, 53, 2240–2249 CrossRef CAS PubMed.
- R. M. Santos, M. S. Rodrigues, J. Laranjinha and R. M. Barbosa, Biosens. Bioelectron., 2013, 44, 152–159 CrossRef CAS PubMed.
- R. Trouillon, M. K. Passarelli, J. Wang, M. E. Kurczy and A. G. Ewing, Anal. Chem., 2013, 85, 522–542 CrossRef CAS PubMed.
- E. A. Hillard, F. C. de Abreu, D. C. M. Ferreira, G. Jaouen, M. O. F. Goulart and C. Amatore, Chem. Commun., 2008, 2612–2628 RSC.
- A. L. Hodgkin and A. F. Huxley, Nature, 1939, 144, 710–711 CrossRef.
- K. M. Gray, E. Kim, L. Q. Wu, Y. Liu, W. E. Bentley and G. F. Payne, Soft Matter, 2011, 7, 9601–9615 RSC.
- J. Sedo, J. Saiz-Poseu, F. Busque and D. Ruiz-Molina, Adv. Mater., 2013, 25, 653–701 CrossRef CAS PubMed.
- E. Faure, C. Falentin-Daudre, C. Jerome, J. Lyskawa, D. Fournier, P. Woisel and C. Detrembleur, Prog. Polym. Sci., 2013, 38, 236–270 CrossRef CAS PubMed.
- J. L. Dalsin, B. H. Hu, B. P. Lee and P. B. Messersmith, J. Am. Chem. Soc., 2003, 125, 4253–4258 CrossRef CAS PubMed.
- H. Lee, J. Rho and P. B. Messersmith, Adv. Mater., 2009, 21, 431–434 CrossRef CAS PubMed.
- H. Lee, S. M. Dellatore, W. M. Miller and P. B. Messersmith, Science, 2007, 318, 426–430 CrossRef CAS PubMed.
- W. E. Bentley and G. F. Payne, Science, 2013, 341, 136–137 CrossRef CAS PubMed.
- H. Ejima, J. J. Richardson, K. Liang, J. P. Best, M. P. van Koeverden, G. K. Such, J. Cui and F. Caruso, Science, 2013, 341, 154–157 CrossRef CAS PubMed.
- S. E. Page, M. Sander, W. A. Arnold and K. McNeill, Environ. Sci. Technol., 2012, 46, 1590–1597 CrossRef CAS PubMed.
- F. Maurer, I. Christl, M. Hoffmann and R. Kretzschmar, Environ. Sci. Technol., 2012, 46, 8808–8816 CrossRef CAS PubMed.
- M. Aeschbacher, C. Graf, R. P. Schwarzenbach and M. Sander, Environ. Sci. Technol., 2012, 46, 4916–4925 CrossRef CAS PubMed.
- D. Del Rio, A. Rodriguez-Mateos, J. P. Spencer, M. Tognolini, G. Borges and A. Crozier, Antioxid. Redox Signaling, 2013, 18, 1818–1892 CrossRef CAS PubMed.
- S. Quideau, D. Deffieux, C. Douat-Casassus and L. Pouysegu, Angew. Chem., Int. Ed., 2011, 50, 586–621 CrossRef CAS PubMed.
- J. McGinness, P. Corry and P. Proctor, Science, 1974, 183, 853–855 CAS.
- J. E. McGinness, Science, 1972, 177, 896–897 CAS.
- G. Milczarek and O. Inganas, Science, 2012, 335, 1468–1471 CrossRef CAS PubMed.
- L. Q. Wu, R. Ghodssi, Y. A. Elabd and G. F. Payne, Adv. Funct. Mater., 2005, 15, 189–195 CrossRef CAS.
- L. Q. Wu, M. K. McDermott, C. Zhu, R. Ghodssi and G. E. Payne, Adv. Funct. Mater., 2006, 16, 1967–1974 CrossRef CAS.
- E. Kim, Y. Liu, X. W. Shi, X. H. Yang, W. E. Bentley and G. F. Payne, Adv. Funct. Mater., 2010, 20, 2683–2694 CrossRef CAS.
- W. Suginta, P. Khunkaewla and A. Schulte, Chem. Rev., 2013, 113, 5458–5479 CrossRef CAS PubMed.
- L. Q. Wu, A. P. Gadre, H. M. Yi, M. J. Kastantin, G. W. Rubloff, W. E. Bentley, G. F. Payne and R. Ghodssi, Langmuir, 2002, 18, 8620–8625 CrossRef CAS.
- J. Redepenning, G. Venkataraman, J. Chen and N. Stafford, J. Biomed. Mater. Res., Part A, 2003, 66, 411–416 Search PubMed.
- R. Fernandes, L. Q. Wu, T. H. Chen, H. M. Yi, G. W. Rubloff, R. Ghodssi, W. E. Bentley and G. F. Payne, Langmuir, 2003, 19, 4058–4062 CrossRef CAS.
- X. L. Luo, J. J. Xu, Y. Du and H. Y. Chen, Anal. Biochem., 2004, 334, 284–289 CrossRef CAS PubMed.
- X. Pang and I. Zhitomirsky, Mater. Chem. Phys., 2005, 94, 245–251 CrossRef CAS PubMed.
- R. A. Zangmeister, J. J. Park, G. W. Rubloff and M. J. Tarlov, Electrochim. Acta, 2006, 51, 5324–5333 CrossRef CAS PubMed.
- Y. Cheng, X. L. Luo, J. Betz, S. Buckhout-White, O. Bekdash, G. F. Payne, W. E. Bentley and G. W. Rubloff, Soft Matter, 2010, 6, 3177–3183 RSC.
- W. Q. Sun, G. F. Payne, M. Moas, J. H. Chu and K. K. Wallace, Biotechnol.
Prog., 1992, 8, 179–186 CrossRef CAS.
- C. Muzzarelli and R. A. A. Muzzarelli, Trends Glycosci. Glycotechnol., 2002, 14, 223–229 CrossRef CAS.
- G. F. Payne, M. V. Chaubal and T. A. Barbari, Polymer, 1996, 37, 4643–4648 CrossRef CAS.
- G. Kumar, J. F. Bristow, P. J. Smith and G. F. Payne, Polymer, 2000, 41, 2157–2168 CrossRef CAS.
- L. Q. Wu, H. D. Embree, B. M. Balgley, P. J. Smith and G. F. Payne, Environ. Sci. Technol., 2002, 36, 3446–3454 CrossRef CAS.
- G. Kumar, P. J. Smith and G. F. Payne, Biotechnol. Bioeng., 1999, 63, 154–165 CrossRef CAS.
- Y. Liu, E. Kim, M. E. Lee, B. Zhang, Y. A. Elabd, Q. Wang, I. M. White, W. E. Bentley and G. F. Payne, Adv. Funct. Mater., 2013 DOI:10.1002/adfm.201301434.
- J. Saiz-Poseu, J. Sedó, B. García, C. Benaiges, T. Parella, R. Alibés, J. Hernando, F. Busqué and D. Ruiz-Molina, Adv. Mater., 2013, 25, 2066–2070 CrossRef CAS PubMed.
- C. M. Aberg, T. H. Chen, A. Olumide, S. R. Raghavan and G. F. Payne, J. Agric. Food Chem., 2004, 52, 788–793 CrossRef CAS PubMed.
- J. L. Kerwin, D. L. Whitney and A. Sheikh, Insect Biochem. Mol. Biol., 1999, 29, 599–607 CrossRef CAS.
- P. Kavanagh and D. Leech, Phys. Chem. Chem. Phys., 2013, 15, 4859–4869 RSC.
- P. O. Conghaile, S. Poller, D. MacAodha, W. Schuhmann and D. Leech, Biosens. Bioelectron., 2013, 43, 30–37 CrossRef PubMed.
- O. Demkiv, O. Smutok, S. Paryzhak, G. Gayda, Y. Sultanov, D. Guschin, H. Shkil, W. Schuhmann and M. Gonchar, Talanta, 2008, 76, 837–846 CrossRef CAS PubMed.
- S. Behera, S. Sampath and C. R. Raj, J. Phys. Chem. C, 2008, 112, 3734–3740 CAS.
- E. Kim, Y. Liu, C. J. Baker, R. Owens, S. Y. Xiao, W. E. Bentley and G. F. Payne, Biomacromolecules, 2011, 12, 880–888 CrossRef CAS PubMed.
- C. H. Foyer and G. Noctor, Antioxid. Redox Signaling, 2013, 18, 2087–2090 CrossRef CAS PubMed.
- B. Frei, I. Birlouez-Aragon and J. Lykkesfeldt, Crit. Rev. Food Sci. Nutr., 2012, 52, 815–829 CrossRef CAS PubMed.
- E. Kim, Y. Liu, W. E. Bentley and G. F. Payne, Adv. Funct. Mater., 2012, 22, 1409–1416 CrossRef CAS.
- A. Price-Whelan, L. E. P. Dietrich and D. K. Newman, Nat. Chem. Biol., 2006, 2, 71–78 CrossRef CAS PubMed.
- J. J. Chen, W. Chen, H. He, D. B. Li, W. W. Li, L. Xiong and H. Q. Yu, Environ. Sci. Technol., 2013, 47, 1033–1039 CrossRef CAS PubMed.
- L. E. P. Dietrich, A. Price-Whelan, A. Petersen, M. Whiteley and D. K. Newman, Mol. Microbiol., 2006, 61, 1308–1321 CrossRef CAS PubMed.
- A. Price-Whelan, L. E. P. Dietrich and D. K. Newman, J. Bacteriol., 2007, 189, 6372–6381 CrossRef CAS PubMed.
- L. E. P. Dietrich, C. Okegbe, A. Price-Whelan, H. Sakhtah, R. C. Hunter and D. K. Newman, J. Bacteriol., 2013, 195, 1371–1380 CrossRef CAS PubMed.
- L. E. P. Dietrich, T. K. Teal, A. Price-Whelan and D. K. Newman, Science, 2008, 321, 1203–1206 CrossRef CAS PubMed.
- A. Price-Whelan, L. E. P. Dietrich and D. K. Newman, Nat. Chem. Biol., 2006, 2, 71–78 CrossRef CAS PubMed.
- L. K. Branski, A. Al-Mousawi, H. Rivero, M. G. Jeschke, A. P. Sanford and D. N. Herndon, Surg. Infect., 2009, 10, 389–397 CrossRef PubMed.
- B. A. Pruitt, Jr, A. T. McManus, S. H. Kim and C. W. Goodwin, World J. Surg., 1998, 22, 135–145 CrossRef.
- E. E. Tredget, H. A. Shankowsky, R. Rennie, R. E. Burrell and S. Logsetty, Burns, 2004, 30, 3–26 CrossRef PubMed.
-
A. J. Bard and L. R. Faulkner, Electrochemical Methods: Fundamentals and Applications, Wiley, 2000 Search PubMed.
- O. Bukelman, N. Amara, R. Mashiach, P. Krief, M. M. Meijler and L. Alfonta, Chem. Commun., 2009, 2836–2838 RSC.
- D. V. Vukomanovic, D. E. Zoutman, G. S. Marks, J. F. Brien, G. W. van Loon and K. Nakatsu, J. Pharmacol. Toxicol. Methods, 1996, 36, 97–102 CrossRef CAS.
- H. Yang, Curr. Opin. Chem. Biol., 2012, 16, 422–428 CrossRef CAS PubMed.
- K. Ino, T. Nishijo, T. Arai, Y. Kanno, Y. Takahashi, H. Shiku and T. Matsue, Angew. Chem., Int. Ed., 2012, 51, 6648–6652 CrossRef CAS PubMed.
- M. Sen, K. Ino, H. Shiku and T. Matsue, Lab Chip, 2012, 12, 4328–4335 RSC.
- E. Kim, T. Gordonov, W. E. Bentley and G. F. Payne, Anal. Chem., 2013, 85, 2102–2108 CrossRef CAS PubMed.
- E. Kim, T. Gordonov, Y. Liu, W. E. Bentley and G. F. Payne, ACS Chem. Biol., 2013, 8, 716–724 CrossRef CAS PubMed.
- M. G. Espey, Free Radical Biol. Med., 2013, 55, 130–140 CrossRef CAS PubMed.
|
This journal is © The Royal Society of Chemistry 2014 |
Click here to see how this site uses Cookies. View our privacy policy here.