DOI:
10.1039/B809299K
(Paper)
Lab Chip, 2009,
9, 140-144
Long-term and room temperature operable bioactuator powered by insect dorsal vessel tissue†
Received
2nd June 2008
, Accepted 24th September 2008
First published on 21st October 2008
Abstract
We present a bioactuator powered by insect dorsal vessel tissue which can work for a long time at room temperature without maintenance. Previously reported bioactuators which exploit contracting ability of mammalian heart muscle cell have required precise environmental control to keep the cell alive and contracting. To overcome this problem, we propose a bioactuator using dorsal vessel tissue. The insect tissue which can grow at room temperature is generally robust over a range of culture conditions compared to mammalian tissues and cells. First, we confirm that a dorsal vessel tissue of lepidoptera larva Ctenoplusia agnata contracts spontaneously for at least 30 days without medium replacement at 25 °C. Using the dorsal vessel tissue cultured under the same conditions, we succeed in driving micropillars 100 µm in diameter and 1000 µm in height for more than 90 days. The strongest displacement of the micropillar top occurs on the 42nd day and is 23 µm. Based on these results, the contracting force is roughly estimated as 4.7 µN which is larger than that by a few mammalian cardiomyocytes (3.4 µN). Definite displacements of more than 10 µm are observed for 58 days from the 15th to the 72nd days. The number of life cycles can be roughly calculated as 7.5 × 105 times for the average frequency of about 0.15 Hz, which is no less than that of conventional mechanical actuators. These results suggest that the insect dorsal vessel tissue is a more promising material for bioactuators used at room temperature than other biological cell-based materials.
Introduction
Use of real muscle tissues for a linear actuator in a microspace has attracted interest because muscle tissues are superior to artificial muscle tissues.1 Muscle tissues are powered by chemical energy such as glucose. A number of bioactuators using mammalian cardiomyocytes have already been reported, e.g. pillar bioactuators,2,3 a micro heart pump,4,5 a self-assembled microdevice,6 a muscular thin film,7 and other microdevices.8–12 However, these devices require precise environmental control to keep the cardiomyocytes contracting spontaneously. The medium must be replaced every few days and pH and temperature must be kept around 7.4 and 37 °C, respectively. Namely, the bioactuators reported previously have the disadvantage that they can work only when set in a CO2 incubator.
In general, insects and their tissues are surprisingly robust over a much wider range of living conditions as compared to mammals. For instance, fruit flies can tolerate a vacuum. When kept at 55 mTorr pressure for 60 min more than half of the flies survived.13 As another example, a segment of dorsal vessel (DV) tissue of a cockroach embryo continued to contract for more than 260 days in culture medium.14 Due to their robustness not only at an individual level but also at a tissue level, insects could be useful as a biological part of a hybrid robot system. However, there have been only a few studies which utilized an entire insect or insect tissue as a robot part. As examples, a living cockroach was controlled by applying artificial electrical stimuli to its nervous system15 and the antennae of a silk worm were used as a sensor for a pheromone searching robot.16–18
The cell culture techniques for insects are less advanced than for mammals though the first insect cell line was established from a moth in 1962 by Grace.19 Grace insect medium formulated in that study is extensively utilized even now since the numbers of insect media that have been developed are many less than the numbers of mammalian media. As far as muscle tissue is concerned, only the cell line NISES-AnPe-426 was established which formed contractile tissue networks in vitro.20 Unfortunately, this cell line is not available now. Though we have already reported on primary culturing of DV (tubular insect heart) cells, in which some cells migrated from DV tissues of a lepidoptera larva kept contracting spontaneously for more than 18 days at room temperature,21 the mass culturing of contracting cells has not been realized yet; this is necessary for fabricating mechanical components made of insect cells.
In this paper, we propose to utilize not insect cells but insect tissues as a mechanical component and we demonstrate the first example of a micro bioactuator driven by DV tissue. This driving occurs autonomously at room temperature for a long time without maintenance. For this purpose, DV tissue excised from a lepidopteran larva is attached to micropillars and cultured in medium. To evaluate the mechanical properties of the DV tissue, the displacement of a micropillar top is analyzed and the contracting force is estimated from it. Based on these results, the DV tissue is compared to mammalian cardiomyocytes regarding function.
Experimental
DV tissue culture
The number of lepidopteran cell lines has been increasing at 50 lines per decade22 and the culture techniques for lepidopteran cells are more developed compared to cells of other insects. Lepidopteran larvae, Ctenoplusia agnata (CA) which had been bred continuously with only an artificial diet at 25 °C23 were used in this study. Their DVs were excised under stereomicroscopy (ZMM-45B2, Sigma Koki) after surface sterilization in 70% ethanol solution.24 The excised DVs were cut into small pieces and cultured in TC-10025 (Sigma-Aldrich) medium supplemented with 20% fetal bovine serum FBS (Gibco BRL), 5% CA hemolymph (HL), and 1% penicillin-streptomycin solution (Gibco BRL) at 25 °C. The hemolymph was supplemented after being inactivated at 56 °C for 30 min.26 DV tissues were observed under an inverted microscope (IX-71, Olympus) with phase contrast.
Fabrication of a micropillar actuator
The micropillars were fabricated by molding of polydimethylsiloxane (PDMS; Sylpod184, Dow Corning) (Fig. S1 in the ESI).† The mold was fabricated by drilling a 11 × 11 square lattice of through holes with a 100 µm diameter and 300 µm pitch through a 20-square-mm poly(tetrafluoroethylene) PTFE sheet (thickness: 1 mm). The procedure for attaching DV tissue to the micropillars is as follows. First, the film was treated with oxgen plasma to hydrophilize the surface using a quick coater apparatus (SC-701, Sanyu Electron) and then the film was put into a 35 mm diameter petri dish. Second, the petri dish and its contents were sterilized using a UV lamp for 30 min in a clean bench. Next the film was coated with polyphenolic proteins extracted from Mytilus edulis (marine mussel) Cell-Tak (BD Falcon) according to the manufacturer's manual. The DV tissues of two larvae per petri dish were cut into pieces using a pair of scissors and the pieces were stuck onto the micropillars by using forceps. Finally, 3 mL of the culture medium were gently poured into the petri dish and the dish was sealed with parafilm to prevent the medium from evaporating. The micropillars with DV tissue were incubated at 25 °C without medium replacement.
Image analysis for micropillar actuation
The micropillar actuation was evaluated using displacement of a micropillar top. The micropillars were observed with a stereomicroscope (MVX10, Olympus) and the microscopic image was captured with a CCD camera (WAT-221S, Watec) into a PC with AVI format (30 frames/s). The video was converted into a stacked Tiff file with 256 gray scale, which was analyzed with “Scion Image” software (Scion Corp.). To recognize the shape of the micropillar top using the software, black and white in the file were inverted and a threshold was set. Finally, the X–Y coordinate values and the displacements of the center of gravity were calculated by a macro function.
Results and discussion
Culturing of DV tissue
Some pieces of DV tissue kept contracting spontaneously and vigorously at around 0.2 Hz during the first 10 days under the culture conditions mentioned above. The entire piece of DV tissue did not contract simultaneously and each part contracted separately at different frequencies (Fig. S2 and Movie S1 in the ESI).† The contractions continued for more than 30 days without medium replacement though they became weaker and weaker as time passed. It was confirmed that DV tissue retained its spontaneous contractions for more than 30 days with only 500 µl culture medium initially provided at 25 °C. These culture conditions were used in the following experiments.
Micropillar actuation by DV tissue
An actual image of the micropillars with some pieces of DV tissue attached is shown in Fig. 1a,b. No micropillars were actuated and the DV tissue just contracted by itself immediately after plating. The micropillars had been actuated since the 2nd day and a piece of DV tissue actuated several micropillars. The actuated directions and displacements varied depending on micropillars (Fig. 1c and Movie S2 in the ESI).† The micropillar actuated most vigorously on the 2nd day was selected and it was observed and analyzed every 14 days or less throughout the experiment to evaluate the time course of displacement (Fig. 2). The selected micropillar was actuated most vigorously throughout the experiment. The small actuation of less than 2 µm at around 2 Hz and the large actuation of more than 5 µm at around 0.2 Hz were observed on the 2nd day, but the former disappeared in 2 or 3 days. The latter displacement increased as the number of culture days passed and it exceeded 10 µm from the 15th day. The strongest one was observed on the 42nd day (Movie S3 in the ESI)† and it was 23 µm based on comparison of the not-actuated position and the actuated position in the video grab images (Fig. S2 in the ESI).† Definite displacements of more than 10 µm were observed until the 72nd day and they became smaller and smaller as time passed until they were below 7 and 5 µm on the 86th and the 91st days, respectively. No actuation was observed on the 100th day. The same experiments were conducted several times and in most cases the micropillar actuation was observed for approximately 90 days when the DV had been excised and assembled onto the micropillar array without any damage.
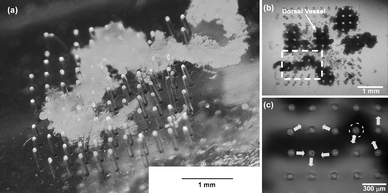 |
| Fig. 1 Actual images of the micropillars with attached DV tissue. (a) Digital microscopy image (KH-7700, Hirox) immediately after assembling. (b) Pieces of DV tissue from two larvae were plated onto the micropillars. The tissue piece in the bottom left contacted and actuated micropillars vigorously. (c) An enlarged view of the area marked by the dotted white rectangle in Fig. (b). Eight micropillars were actuated in various directions as shown by the white arrows on the 15th day (ESI Movie 2).† The micropillar enclosed by the dotted white circle was actuated most vigorously and it was selected for observation from the 2nd day until the end of the experiment. | |
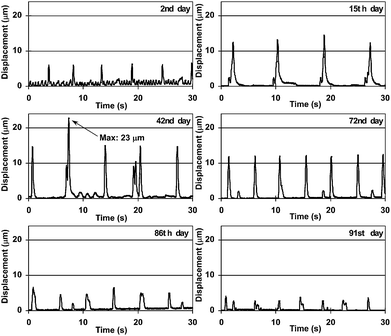 |
| Fig. 2 Image analysis results of micropillar displacement. The time courses of the displacement of the same micropillar top are shown on each day. | |
Actuating direction of the micropillar
The micropillar selected for observation was not always actuated in the same direction with the same displacement. Stronger displacements than usual were occasionally observed. The time course of the displacement and the position of the center of gravity on the X–Y plane on the 35th day are plotted in Fig. 3. As a result, the vector of the strongest displacement γ was almost the same as the resultant vetor of the two different directional vectors of α1 and β. Within a living body, the entire DV does not contract simultaneously. The DV is divided into several contracting sections that contract peristaltically in order from the posterior to the anterior; in this way, hemolymph is circulated. The occasional stronger displacement could be explained as simultaneous actuations by two different sections. This showed that contracting direction and displacement depended on positional relationship between contracting sections and a micropillar, and this positional relationship should be considered to utilize DV as an actuator.
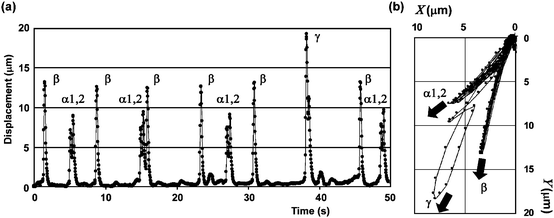 |
| Fig. 3 (a) Time course of displacement of the center of gravity and (b) center of gravity position on the X–Y plane. The graphs were obtained for a 50 s period on the 35th day. The displacements were classified into 4 types: α1, α2, β, γ according to amplitudes, directions, and frequencies in both graphs. α2 always occurred following α1 and was larger than α1 except when α1 and β occurred simultaneously at 38 s in graph (a), but they were independent of β. The displacement of β was larger than those of α1 and α2. A much stronger displacement of γ than those of α1, α2, and β appeared occasionally. The averages of the vectors of α1, α2, and β were (4.6, 6.0), (5.9, 7.2), and (3.2, 12.5), respectively, and the vectors of γ were (7.7, 17.7). The vectors of γ almost agreed with the resultant vector of the vectors of α1 and β. Therefore, the occasional stronger displacements could be explained as simultaneous actuations to two different directions. | |
Long time durability of insect micropillar actuator
The time courses of the frequency of displacements of more than 10 µm and the strongest displacement are shown in (Fig. 4). Definite displacements of more than 10 µm were observed for 58 days from the 14th day to the 71st day. The frequencies remained at 0.13 Hz or more after the 14th day. Hence, the number of cycles of this actuator could be calculated roughly as 7.5 × 105 when the average frequency was 0.15 Hz, which would be enough for practical use and was similar to that of conventional mechanical actuators: piezoelectric, 106 to 108; shape memory alloy, 105; polymer, 105.1
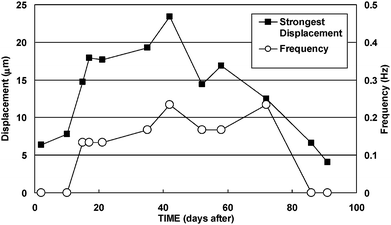 |
| Fig. 4 Time courses of the frequency and the strongest displacement. The plotted frequencies did not include actuations less than 10 µm on each day. Definite displacements of more than 10 µm were observed for 58 days from the 15th day to the 72nd day. Therefore, the number of life cycles of this micropillar actuator could be calculated as 7.5 × 105 as the average frequency was 0.15 Hz. | |
Estimation of magnitude of the contracting force of DV tissue
Proposals have already been made to estimate the cellular traction or contracting force using micropillars.27,28 In order to evaluate the performance of the DV tissue actuator and compare it with a cardiomyocyte actuator, the contracting force of DV was estimated in a similar manner. The estimation model is shown in Fig. 5a. As the angle of inclination θ is small, it can be treated as an ideal elastic solid and sinθ can be approximated by θ. Total displacement D is represented by
where D1 and D2 are the respective displacements below and above where the DV tissue is attached, P is contracting force, and E is Young's modulus for PDMS which is 2.5 MPa,27I is geometrical moment of inertia πd4/64 (d: diameter of the micropillar, 0.05 mm), X is height at which a DV tissue attaches to a micropillar, and L is micropillar height, 1mm. Hence, the contracting force is given by
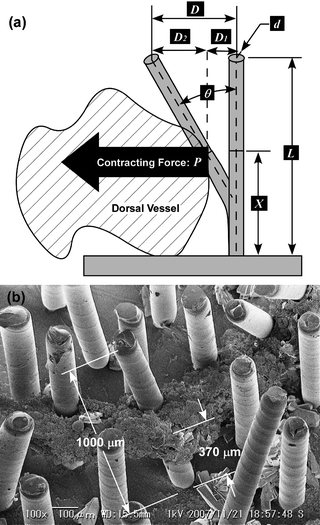 |
| Fig. 5 Estimation of the contracting force of a DV tissue. Parameters to calculate the driving force are shown in (a). X = 370 µm was obtained by (b) SEM observation of the micropillar which had been selected on the 2nd day. | |
To measure the height X, the DV tissues with attached micropillars were observed with SEM after tissue fixation: they were fixed with 4% paraformaldehyde in 0.1 M phosphate buffer, dehydrated through a graded alcohol series (70, 80, 90, and 100% ethanol for 5 min once each), and freeze-dried with t-butyl alcohol. Based on the SEM measurement results (Fig. 5b), the height X was 370 µm when the contracting force was applied to the center point of the attaching area. By substituting D for 23 µm, the contracting force P = 4.7 µN was obtained. However, the actual contracting force must be in excess of the estimated force in this study. The DV can be considered to shorten completely since a PDMS micropillar with a high aspect ratio (height/diameter) of 10 is very deformable and the displacement of the most contracting section of DV was 30 µm or less based on our observation. If moderately stiff micropillars are used, the estimated contracting force may be larger than in the present paper and it may be closer to the contracting force of a DV tissue. Furthermore, the contracting force estimated from the displacement of a micropillar top was probably underachieving since the micropillar was pulled from one or more directions as already mentioned.
Comparison of insect DV tissues and mammalian cardiomyocytes
An insect DV tissue is compared in Table 1 with mammalian cardiomyocytes which is the leading candidate for a bioactuator. The DV tissue retained the ability for spontaneous contractions in the initially provided culture medium of 1 mL per a DV at 25 °C. Definite actuation continued for 58 days from the 15th day to the 72nd day. On the other hand, mammalian cardiomyocytes needed to have the medium replaced every few days and suitable culture conditions (37 °C and 5 % CO2 atmosphere) had to be controlled; but even then contraction stopped in around 6 days.9 Thus, the DV tissue required little maintenance to keep its viability, which would be an advantage in the application as a mechanical part. Furthermore, the contracting force estimated from the displacement was 4.7 µN, which was larger than 3.5 µN reported by Tanaka et al.3 These results suggested that the DV tissue was strong enough to drive a micro mechanical part since some hybrid devices actuated by cardiomyocytes have already been reported. From the viewpoint of the difference between them, mammalian cardiomyocytes are connected with each other by gap junctions and contract simultaneously.29 On the other hand, the DV tissue was divided into some contracting sections and it did not contract simultaneously. Though this non-synchronization would be less desirable for exploiting a strong actuation, it could be suitable for peristaltic pumping.
Table 1 Properties of insect DV tissue and mammalian cardiomyocytea
|
Lifetime |
Frequency |
Number of life cycles |
Contracting force |
Medium replacement |
Temperature |
The insect DV tissue was superior to the cardiomyocyte except for the frequency. It is notable that the DV tissue does not need medium replacement and heating to 37 °C. The values obtained by Tanaka et al.3 or Kim et al.9 were used as the lifetime, frequency, and contracting force of the cardiomyocyte tissue. The number of life cycles was calculated by multiplying the lifetime by the frequency.
|
Insect DV tissue |
58 days |
0.15 Hz |
7.5 × 105 |
4.7 µN |
Not Needed |
25 °C |
Mammalian cardiomyocyte |
6 days |
1 Hz |
5.2 × 105 |
3.5 µN |
2–3 days |
37 °C |
Conclusions
We succeeded in actuating micropillars autonomously using spontaneous contraction of DV tissue. The actuations continued for more than 90 days at 25 °C without medium replacement. The strongest displacement was 23 µm on the 41st day. The contracting force estimated from the strongest displacement was 4.7 µN. The displacement and the contracting force were larger than the respective values of 2.8 µm and 3.8 µN for mammalian cardiomyocytes, though the micropillar size was different for the two. From the viewpoint of durability, the number of life cycles could be calculated as over 1.6 × 106 as the micropillar was actuated at an average frequency of 0.2 Hz for more than 90 days. This was not inferior to the performance of conventional artificial actuators. These results suggested that DV tissue is not only more efficient and powerful but also more environmentally robust as a mechanical part for a bioactuator compared to mammalian cardiomyocytes. Though the present paper only described a demonstration of the principle and performance of a primitive micropillar actuator, we think the insect tissue will be applied for various hybrid devices in the future.
As an application for other fields, this micropillar actuator can be utilized as a research tool for insect physiology. Current studies on insect DVs depend on conventional electrophysiological experiments using micro glass needles as microelectrodes, which is not suitable for long-term monitoring and cannot assess mechanical properties. This evaluation technique using micropillars could enable researchers to evaluate the mechanical properties of DVs long-term without damage and is particularly useful for investigating the effects of biologically active agents such as neuropeptides.
Acknowledgements
The present work was supported by Grants-in-Aid for Scientific Research from the Ministry of Education, Culture, Sports, Science and Technology in Japan Nos. 18656042 and 19016008, the Industrial Technology Research Grant Program (2006) from New Energy and Industrial Technology Development Organization (NEDO) of Japan and the Sasakawa Scientific Research Grant. Fruitful discussions with Dr. Mitsuhashi and Dr. Imanishi of National Institute of Agrobiological Sciences (NIAS) about insect cell culturing are gratefully acknowledged. Thanks are expressed to Mr. Yamamoto in Iwabuchi Laboratory, Tokyo University of Agriculture and Technology for providing lepidopteran insects.
References
- I. W. Hunter and S. Lafontaine, Technical Digest. IEEE Solid-State Sensor and Actuator Workshop, 1992, 178–185 Search PubMed.
- K. Morishima, Y. Tanaka, M. Ebara, T. Shimizu, A. Kikuchi, M. Yamato, T. Okano and T. Kitamori, Sens. Actuators, B, 2006, 119, 345–350 CrossRef.
- Y. Tanaka, K. Morishima, T. Shimizu, A. Kikuchi, M. Yamato, T. Okano and T. Kitamori, Lab Chip, 2006, 6, 230–235 RSC.
- Y. Tanaka, K. Morishima, T. Shimizu, A. Kikuchi, M. Yamato, T. Okano and T. Kitamori, Lab Chip, 2006, 6, 362–368 RSC.
- Y. Tanaka, K. Sato, T. Shimizu, M. Yamato, T. Okano and T. Kitamori, Lab Chip, 2007, 7, 207–212 RSC.
- J. Xi, J. J. Schmidt and C. D. Montemagno, Nat. Mater., 2005, 4, 180–184 CrossRef CAS.
- A. W. Feinberg, A. Feigel, S. S. Shevkoplyas, S. Sheehy, G. M. Whitesides and K. K. Parker, Science, 2007, 317, 1366–1370 CrossRef CAS.
- D. H. Kim, J. Y. Park, K. Y. Suh, P. Kim, S. K. Choi, S. C. Ryu, S. H. Park, S. H. Lee and B. Kim, Sens. Actuators, B, 2006, 117, 391–400 CrossRef.
- J. Kim, J. Park, S. Yang, J. Baek, B. Kim, S. H. Lee, E. S. Yoon, K. Chun and S. Park, Lab Chip, 2007, 7, 1504–1508 RSC.
- J. Park, I. C. Kim, J. Baek, M. Cha, J. Kim, S. Park, J. Lee and B. Kim, Lab Chip, 2007, 7, 1367 RSC.
- T. Ishisaka, H. Sato, Y. Akiyama, Y. Furukawa and K. Morishima, IEEE Int.Conf. Solid-State Sensors, Actuators and Microsystems, 2007, 903–906 Search PubMed.
- Y. Akiyama, Y. Furukawa and K. Morishima, IEEE Int.Conf. Engineering in Medicine and Biology, 2006, 6565–6568 Search PubMed.
- A. J. Shum and B. A. Parviz, IEEE Int. Conf. Micro Electro Mechanical Systems, 2007, 179–182 Search PubMed.
- W. Larsen, Life Sciences, 1963, 8, 606–610 CrossRef CAS.
- R. Holzer and I. Shimoyama, IEEE/RSJ Int. Conf. Intelligent Robots and Systems, 1997, 1514–1519 Search PubMed.
- Y. Kuwana, I. Shimoyama and H. Miura, IEEE/RSJ Int. Conf. Intelligent Robots and Systems, 1995, 530–535 Search PubMed.
- Y. Kuwana, S. Nagasawa, I. Shimoyama and R. Kanzaki, Biosens. Bioelectron., 1999, 14, 195–202 CrossRef CAS.
- Y. Kuwana and I. Shimoyama, Int. J. Robot. Res., 1998, 17, 924–933 Search PubMed.
- T. D. C. Grace, Nature, 1962, 195, 788–789 CrossRef CAS.
- H. Inoue, J. Kobayashi, H. Kawakita, J. I. Miyazaki and T. Hirabayashi, In Vitro Cell. Dev. Biol, 1991, 27A, 837–840 CrossRef CAS.
- Y. Akiyama, K. Iwabuchi, Y. Furukawa and K. Morishima, J. Biotechnol., 2008, 133, 261–266 CrossRef CAS.
-
L. Dwight, in Methods in Molecular Biology, ed. D. W. Murhammer, Humana Press, Totowa, 2nd edn., 2007, vol. 338, ch. 6, pp. 117–137 Search PubMed.
- K. Kawasaki, M. Ikeuchi and T. Hidaka, Jpn. J. Appl. Ent. Zool., 1987, 31, 78–80 Search PubMed.
-
J. Mitsuhashi, in Invertebrate Tissue Culture Methods, Springer, Tokyo, 2002, ch. 5, pp. 47–71 Search PubMed.
- G. R. Gardiner and H. Stockdale, J. Invertebr.Pathol., 1975, 25, 363–370 CrossRef.
- S. S. Wyatt, J. Gen. Physiol., 1956, 39, 841–852 CrossRef CAS.
- J. L. Tan, J. Tien, D. M. Pirone, D. S. Gray, K. Bhadriraju and C. S. Chen, Proc. Natl. Acad. Sci. USA, 2003, 100, 1484–1489 CrossRef CAS.
- Y. Zhao and X. Zhang, Sens.Actuator, A, 2006, 125, 398–404 Search PubMed.
- K. Kojima, H. Moriguchi, A. Hattori, T. Kaneko and K. Yasuda, Lab Chip, 2003, 3, 292–296 RSC.
Footnote |
† Electronic supplementary information (ESI) available: Supplementary figures S1–S2 and movies S1–S3. See DOI: 10.1039/b809299k |
|
This journal is © The Royal Society of Chemistry 2009 |