DOI:
10.1039/C6RA10241G
(Paper)
RSC Adv., 2016,
6, 55374-55381
Water soluble poly(styrene sulfonate)-b-poly(vinylidene fluoride)-b-poly(styrene sulfonate) triblock copolymer nanoparticles
Received
20th April 2016
, Accepted 1st June 2016
First published on 2nd June 2016
Abstract
A visible light, Mn2(CO)2-photomediated process was used to enable the iodine degenerative transfer controlled radical polymerization of vinylidene fluoride (VDF) initiated from I–(CF2)6–I, and the successive quantitative activation of the ∼CH2–CF2–I and ∼CF2–CH2–I chain ends of I–PVDF–I, to produce a series of PNpSS-b-PVDF-b-PNpSS triblock copolymers (NpSS/VDF/NpSS = 4/60/4, 15/60/15, 34/60/34) with neopentyl styrene sulfonate (NpSS), which upon NaN3 deprotection, afforded the corresponding PNaSS-b-PVDF-b-PNaSS. All blocks, as well as PVDF, PNpSS and PNaSS formed water stable dispersion/solutions following either nanoprecipitation from acetone (PVDF, PNpSS, and PNpSS-b-PVDF-b-PNpSS) or direct dissolution in water (PNaSS, PNaSS-b-PVDF-b-PNaSS). Remarkably, all PNaSS-b-PVDF-b-PNaSS triblocks also provided indefinitely stable systems even under the high ionic strength conditions of phosphate buffered saline (PBS) solutions, corresponding to cell-isotonic, pH = 7.4 conditions. These trends were found to be consistent with the block composition dependence of the apparent hydrodynamic radius (Rh), conductivity, and zeta potential (ζ), where Rh increases upon deprotection from about 35–25 nm to about 168–136 nm in water, and decreases to 133–86 nm in PBS solutions, ζ decreases from ∼−40 mV to ∼−70 mV in water and increases to ∼−18 mV in PBS, and where the conductivity is negligible for PNpSS-b-PVDF-b-PNpSS but then increases linearly to ∼0.2 mS cm−1 for PNaSS-b-PVDF-b-PNaSS in water to reach ∼17 mS cm−1 in PBS. Finally, the blocks were evaluated as promising 19F-MRI contrast agents.
Introduction
Fluorinated polymers derived from main chain fluorinated alkenes including vinylidene fluoride (VDF, CH2
CF2), tetrafluoroethylene, hexafluoropropene, chlorotrifluoroethylene, etc. are a well-marketed,1,2 high-end class of special materials that exhibit great chemical, thermal and weathering resistance, as well as low refractive index, surface energy, moisture absorption and flammability.3,4 These properties make them in great demand for high performance applications including fuel cell membranes/separators, electrolytes,5 O-rings for extreme temperatures, optical fibers, antifouling coatings/paints, pipe liners, transmission fluids, etc. Moreover, poly(vinylidene fluoride) (PVDF) and its random copolymers also exhibit piezo/ferroelectric behaviour, with applications in sensors, transducers, actuators, and high power capacitors etc.6–8
However, the low boiling point (e.g. VDF, bp = −84 °C) and low reactivity of fluoroalkenes typically demand high pressure, high temperature metal reactors,9 and render the corresponding polymerizations challenging on a laboratory scale. As such, the synthesis of well-defined fluoropolymer architectures remains a difficult synthetic target.10,11 Indeed, while conventional monomers (styrene, (meth)acrylates, dienes, etc.) can easily be homo-, co- and block-polymerized in a controlled fashion by either radical12 or anionic mechanisms, using Schlenk tubes at atmospheric pressure, by contrast, the progress in the controlled radical polymerization (CRP) of VDF has been slow,13 even though VDF is the first monomer to be polymerized by CRP on an industrial scale, using the iodine degenerative transfer (IDT)14,15 method, over 30 years ago.16 As such, since until recently10 the synthesis of well-defined PVDF block copolymers was not readily available, a lag between the study of the well-understood blocks of conventional monomers, and those based on main chain fluoroalkenes, still persists.17 To this end, we have started a research effort towards developing convenient, accessible chemistry for the synthesis of well-defined PVDF architectures, employing low pressure glass tubes and photochemical reactions.6,10,18–25
Our initial efforts centred on elaborating the metal mediated synthesis of PVDF in low pressure glass tubes with either Cu26 or Ti18 complexes. However, while transition metal catalysis has proven quite reliable in ATRP (e.g. Cu halides),27 and respectively, in organometalically (e.g. Cp2TiCl2) mediated CRPs of styrene,28,29 dienes,30,31 as well as in ring opening32–34 and grafting35 polymerizations, including those of fluorinated styrenes and acrylates,36 these systems were ineffective in VDF polymerizations.
Inspired by promising results obtained in room temperature VDF free radical polymerization (FRP) initiated by t-butyl peroxide under UV radiation,26 we have investigated and optimized a large number of photopolymerization systems active under visible light, and recently reported the first examples of the VDF free radical and controlled radical (IDT) polymerizations initiated directly from alkyl, semi- and perfluorinated halides (Cl, Br, I) in the presence of transition metal carbonyl complexes (e.g. Mn2(CO)10, etc.)16,19–22,24 as well as from hypervalent iodides (e.g. ((CX3COO)2IPh) X = H, F) alone or with perfluoroalkyl iodides or molecular iodine.20 Besides the demonstration of the CRP with a linear dependence of the molecular weight (Mn) on conversion, a very important outcome of this study10 was the understanding of the conversion dependence of the PVDF–I chain ends (i.e. of the reactive ∼CH2–CF2–I and of the unreactive ∼CF2–CH2–I) in the IDT process, and the demonstration of their quantitative photoactivation by various transition metal carbonyls, towards the synthesis of well-defined AB or ABA block copolymers of VDF with styrene, methyl acrylate, methyl methacrylate, vinyl chloride, vinyl acetate, isoprene, butadiene, acrylonitrile,19 methyl 2-(trifluoromethyl)acrylate and 2,2,2-trifluoroethyl methacrylate21 as well as with neopentyl styrene sulfonate (NpSS) and styrene sulfonate (PVDF-b-PNaSS).22
Apart from the applications described above, fluorinated materials, including block copolymers have a great potential for biomedical applications, as perfluorinated compounds are usually stable at in vivo conditions.37 Indeed, emulsified simple fluorocarbons or partly fluorinated systems can be used in magnetic resonance imaging (MRI) via 19F detection37–42 or as a drug carriers.43–46 The use of more complex fluoropolymer materials47–49 however, is often hindered by their high hydrophobicity, crystalline nature and inertness for some applications. Thus, physical hydrophilic coating or copolymerization with hydrophilic monomers is required for applications in water environments.
As such, micelle forming, side-chain fluorinated ethers were copolymerized,50 while end-functionalization of poly(ethylene oxide) (PEO) with fluorinated compounds allowed the preparation of hydrogels with controlled surface erosion.51 Combination of PEO with fluorocarbons52 and phospholipids allowed preparation of stable micelles with internal fluorous phase53 or formulations of nanometric DNA particles for gene delivery.54 Methacrylic acid copolymers with dodecafluoroheptyl methacrylate afforded water soluble aggregates of various shapes and structures,55 while surfactant-stabilized emulsions of hydrophobic monomers, such as styrene, n-butyl acrylate, 2,2,3,3,4,4,4-heptafluorobutyl acrylate and methyl trifluoroacrylate enabled synthesis of compact, spherical water-based latexes with different sizes.56 However, aside from a few examples including the grafting of ozone-treated PVDF with N-vinyl-2-pyrrolidone and 2-(dimethylamino)ethyl methacrylate,57 acrylic acid,58 or poly(ethylene glycol) methyl ether methacrylate59 to produce wettable, pH-sensitive58 microfiltration membranes with e.g. bactericidal,57 anti-fouling effect, there is little work towards the synthesis of water-soluble PVDF based materials, especially block copolymers. Moreover, the need for the development of novel sulfonated fluoropolymers for fuel cell membranes60 and other applications including the study of their self-assembly,61 still remains.
Following our initial report on the synthesis of PVDF-b-PNpSS and PVDF-b-PNaSS,22 we are presenting herein the synthesis and characterization of ABA-type PNpSS-b-PVDF-b-PNpSS and of their corresponding, hydrophilic PNaSS-b-PVDF-b-PNaSS triblock copolymers of various compositions and molecular weights, towards the preparation of water stable nanoparticles in a biologically relevant medium, such as phosphate buffered saline solutions with pH ∼ 7.4.
Results and discussion
The preparation of ABA vs. AB block copolymers is preferred due to the beneficial effect of di- vs. monofunctional perfluoroalkyl iodide initiators used in the Mn2(CO)10-mediated IDT of VDF. Indeed, such difunctional I–RF–I minimize the effect of termination and transfer side reactions in IDT and provide I–PVDF–I with high iodine chain end functionality, suitable for the synthesis of block copolymers.10 The synthesis of PNpSS-b-PVDF-b-PNpSS with various compositions is demonstrated by 1H and 19F NMR in Fig. 1 and 2. While this particular I–PVDF–I sample contained predominantly the less reactive ∼CH2–I termini, the complete activation of both ∼CH2–CF2–I and ∼CF2–CH2–I chain ends of PVDF is confirmed by the disappearance of the corresponding resonances c and c′ (δ = 3.62 and 3.87 ppm, 1H-NMR, Fig. 1) and respectively of c, c1, c2 (−39.5, −94.2 and −40.3 ppm) and c′, c′1 (−109.3 ppm and 113.1 ppm) in 19F-NMR Fig. 2. Likewise, the formation of the blocks is established by the PNpSS resonances e, f, g, h, i, j (1H-NMR, Fig. 1), which also enable the calculation of the block copolymer composition, as presented in Table 1, as well as by the new a′3, a′4 and a′5 peaks, which demonstrate10 the PVDF–PNpSS connectivity (19F-NMR Fig. 2).
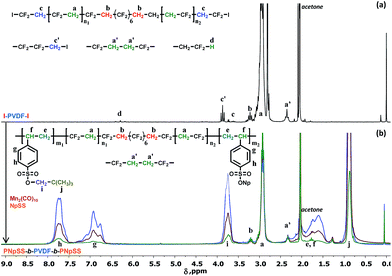 |
| Fig. 1 1H NMR spectra of (a) I-PVDF-I macroinitiator. (b) T1, T2 and T3 PNpSS-b-PVDF-b-PNpSS triblock copolymers. | |
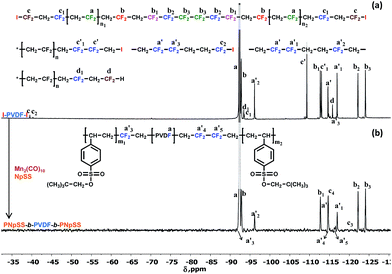 |
| Fig. 2 19F NMR spectra of (a) I–PVDF–I macroinitiator and (b) PNpSS-b-PVDF-b-PNpSS triblock T3. | |
Table 1 Characterization of PVDF, PNpSS, and PNpSS-b-PVDF-b-PNpSS
Exp |
Polymer |
Compositiona NpSS/VDF/NpSS |
Mnb |
PDIb |
Mna |
% NpSSa |
From 1H NMR. from GPC. |
1 |
PVDF |
— |
6200 |
1.43 |
4400 |
0 |
2 |
PNpSS |
— |
7800 |
1.20 |
— |
100 |
3 |
T1 |
4/60/4 |
8900 |
1.26 |
6400 |
11.8 |
4 |
T2 |
15/60/15 |
16 000 |
1.49 |
12 000 |
33.3 |
5 |
T3 |
34/60/34 |
13 800 |
1.86 |
21 700 |
56.5 |
The suitability of the Mn2(CO)10-photomediated chemistry in the synthesis of novel PNpSS-b-PVDF-b-PNpSS triblock copolymers was additionally confirmed by the GPC results (Fig. 3), which indicate a continuous increase in the molecular weight of the copolymers which scales directly with the increasing the PNpSS content while retaining acceptable polydispersity values. Moreover, while PVDF typically presents a negative peak in RI detectors, the progressively larger PNpSS blocks gradually shift the signal to positive values.
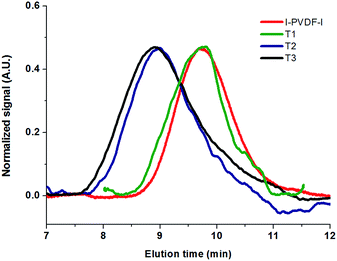 |
| Fig. 3 GPC chromatograms of PVDF, T1, T2 and T3. The signals of I–PVDF–I and T1 are multiplied with −1 and the intensities of all signals are normalized for comparison. | |
The quantitative deprotection of the neopentyl group in the presence of NaN3 and formation of the sodium sulfonate salt is subsequently demonstrated (NMR) in Fig. 4 by the disappearance of the characteristic –CH2– and –CH3 resonances (peaks i and j at δ = 3.69 ppm and 0.77 ppm) of the neopentyl group. PNpSS and its corresponding PNaSS homopolymers were prepared similarly to the triblocks and used for comparison purposes.
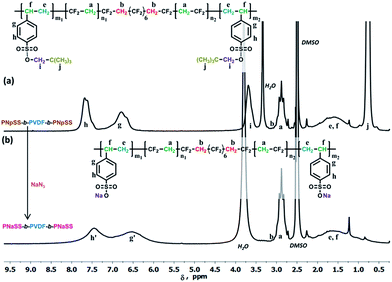 |
| Fig. 4 (a) 1H NMR (DMSO-d6) spectra of (a) P(NpSS)34–P(VDF)60–P(NpSS)34 (triblock T3) and (b) P(NaSS)34–P(VDF)60–P(NaSS)34 (triblock T3D). | |
Since I–PVDF–I, PNpSS as well as all PNpSS-b-PVDF-b-PNpSS triblocks were soluble in acetone, the corresponding nanoparticle solutions were prepared by nanoprecipitation.62 Conversely, following deprotection, the PNaSS homopolymer and all PNaSS-b-PVDF-b-PNaSS triblocks became insoluble in acetone. While they remained soluble in DMSO and DMAC, such high boiling solvents are inappropriate for nanoprecipitation with subsequent evaporation of the organic volatiles. Remarkably, the PNaSS-b-PVDF-b-PNaSS triblocks were found to be water soluble and their 1 mg mL−1 solutions, as well as that of PNaSS were thus directly prepared and studied. In all cases, the water dispersions and solutions were stable for months.
Even more remarkable behaviour was observed upon testing the stability of the nanoparticles and solutions at physiological conditions (0.14 M NaCl) of high ionic strength. By nanoprecipitation into a PBS solution, or by dilution of the stable, water-based nanoprecipitated systems with a twice-concentrated PBS stock solution, all NpSS based polymers as well as I–PVDF–I were unstable and flocculated. Conversely, the deprotected PNaSS based block polymers maintained their long term stability after applying PBS. The solutions were subsequently studied by evaluating the dependence of the polymer apparent hydrodynamic radii (Rh), conductivity and zeta potential (ζ) on composition. These results are summarized in Table 2, where the zeta potential and conductivity values for the pure PBS buffer are also provided for comparison.
Table 2 Characterization of polymer solutionsa
Entry |
Rh [nm] |
ζ-potential [mV] |
Conductivity [mS cm−1] |
% SS |
water |
PBS |
water |
PBS |
water |
PBS |
The missing values of I–PVDF–I, PNpSS, T1, T2 and T3 respectively in PBS indicate macroprecipitation of polymers. |
PVDF |
108 |
— |
−33.8 |
— |
0.0039 |
— |
0 |
T1 |
35 |
— |
−47.7 |
— |
0.0161 |
— |
11.8 |
T2 |
34 |
— |
−42.0 |
— |
0.0101 |
— |
33.3 |
T3 |
25 |
— |
−39.8 |
— |
0.0090 |
— |
56.5 |
T1D |
168 |
133 |
−62.5 |
−24.4 |
0.0315 |
17.1 |
11.8 |
T2D |
166 |
97 |
−43.8 |
−16.6 |
0.1150 |
17.1 |
33.3 |
T3D |
136 |
86 |
−73.6 |
−18.1 |
0.1590 |
17.4 |
56.5 |
PNpSS |
— |
— |
−29.7 |
— |
0.0191 |
— |
100 |
PNaSS |
— |
— |
−60.5 |
— |
0.2140 |
— |
100 |
PBS |
— |
— |
— |
−17.4 |
— |
16.4 |
— |
The dependence of Rh on block composition is presented in Fig. 5. Since these are not single chain nanoparticles, the dependence of Rh on composition and nature of the block (PNpSS vs. PNaSS) is also influenced by the number of chains/particle, which will change upon deprotection, varying the block composition, or the nanoparticle preparation method. While this dependence is complex, and beyond the scope of this study, the comparison between the various systems still remains meaningful, and the observed trends are as follows.
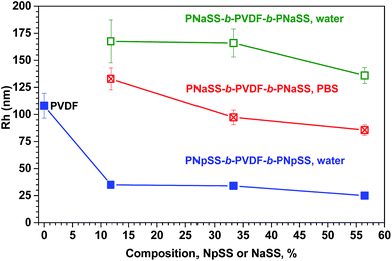 |
| Fig. 5 Dependence of the apparent hydrodynamic radius (Rh) on block copolymer composition. | |
While the starting I–PVDF–I affords aggregates with Rh = 108 nm, block copolymerization leads to a large decrease in the size of nanoparticles to about 35 nm even for only ∼12% NpSS. Furthermore, although the block molecular weight increases continuously, Rh remains relatively constant (35–34 nm) while tripling the % PNpSS from 11.8 to 33.3%, and decreases only slightly (35 to 25 nm) with further increasing the PNpSS content from 33.3 to 56.5%. Likewise, while the deprotection to the hydrophilic, ionic –SO3Na moieties prompts the expansion of the macromolecular coils and a dramatic increase in the Rh of the PNaSS-b-PVDF-b-PNaSS nanoparticles to about 170 nm, a similar weak dependence on composition (168 to 166 to 136 nm) is observed.
The addition of PBS into the –SO3Na systems increases the ionic strength and screens electrostatic interactions, causing the typical shrinkage of polyelectrolytes via counterion condensation along the polymer backbone.63–66 As such, the PNaSS-b-PVDF-b-PNaSS nanoparticles shrink by ∼20%, 60% and 40% to respectively 133, 97 and 86 nm. The composition dependence of the zeta potential and conductivity of these systems is outlined in Fig. 6 and 7 and helps explain the stability of the polymeric nanoparticles.
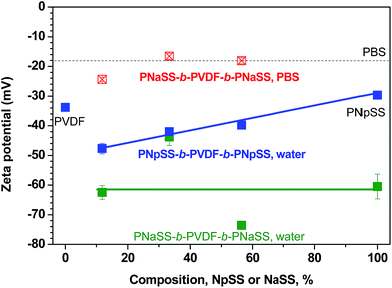 |
| Fig. 6 Dependence of the zeta potential (ζ) on block copolymer composition. The lines are added to help the eye follow the trends. | |
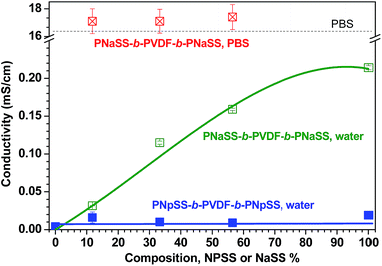 |
| Fig. 7 Dependence of conductivity on block copolymer composition. | |
Indeed, a larger absolute value of ζ indicates stronger electrostatic repulsion between particles, and thus correlates with an increase in the stability of colloidal dispersions. Interestingly, I–PVDF–I (Fig. 6) already displays a highly negative ζ value of ∼−34 mV. This is most likely a consequence of the highly polar C–F bonds.
Upon adding a small PNpSS block (∼12%), ζ decreases to −48 mV indicating a synergistic effect between PVDF and PNpSS. However, further increases in the PNpSS block length lead to a linear increase of ζ to ∼−30 mV for pure PNpSS, a value similar to pure PVDF. This is consistent with the self-assembly of these copolymers in water, where the PNpSS block is slightly more hydrophilic/polar than PVDF. Thus, at higher NpSS compositions, the PVDF core is buried, and ζ is primarily determined by polarity of Ph–SO2–O–CH2–C(CH3)3 structures.
Upon deprotection, due to the more polar –SO3Na groups in the triblock polyelectrolyte, ζ decreases to about −60 mV. The scattering of the ζ values for the intermediate blocks is likely caused by electrolytic effect of high voltage applied to a highly ionized polyelectrolyte during the measurement. Conversely, the PBS buffer drastically reduces the zeta potential for all copolymers to values ζ = −24 mV to −16 mV. This is caused by high concentration of PBS salts, shielding charges of the polyelectrolyte, as indeed the obtained ζ values are close to the pure PBS background (ζ = −17.4 mV).
The corresponding conductivity measurements are presented in Fig. 7. An almost negligible conductivity, commensurate with the lack of charge carriers is seen for PVDF, PNpSS and their block copolymers in water and may originate from traces of inorganic salts (Mn) still present in the prepared material. Deprotection, and thus availability of sodium and styrene sulfonate ions increases conductivity of water solutions in an almost linear fashion with composition, towards the plateau values of PNaSS (∼0.2 mS cm−1). In the presence of the buffer, the conductivity of the PNaSS-b-PVDF-b-PNaSS (17.1 to 17.4 mS cm−1) blocks appears even larger than, or at least similar to that of the baseline PBS (16.4 mS cm−1).
Finally, a proof-of-concept example of the use of the PSSNa-b-PVDF-b-PSSNa triblock systems (T1D, 3 mg mL−1 solution) in 19F-MRI imaging is presented in Fig. 8. As PVDF itself is rather crystalline, it does not provide a strong 19F NMR signal in aqueous media. However, upon swelling with H2O/DMSO, a signal strong enough for 19F-MRI can be acquired. This specific behaviour will allow in situ observation of drug loading and release, and is currently under investigation.
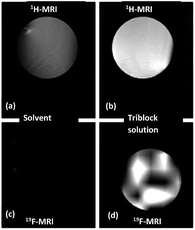 |
| Fig. 8 1H (top row) and 19F (bottom row) phantom images of water–DMSO (2 : 1) solution of PSSNa-b-PVDF-b-PSSNa T1D triblock, 3 mg mL−1. Left: Solvent reference. Right: Polymer solution. | |
Conclusions
The Mn2(CO)2 photomediated iodine degenerative transfer controlled radical polymerization of vinylidene fluoride initiated from I–(CF2)6–I was used to prepare difunctional I–PVDF–I with high (90%) chain end functionality. Subsequent quantitative photoactivation of both –CH2–CF2–I and –CF2–CH2–I chain ends of I–PVDF–I by Mn2(CO)2 in the presence of neopentyl styrene sulfonate (NpSS) led to a series of novel PNpSS-b-PVDF-b-PNpSS triblock copolymers (NpSS/VDF/NpSS = 4/60/4, 15/60/15, 34/60/34), which, upon deprotection with NaN3 afforded the corresponding PNaSS-b-PVDF-b-PNaSS, as confirmed by 1H and 19F NMR, and GPC. All blocks, as well as I–PVDF–I and PNpSS were found to provide water stable dispersion/solutions following either nanoprecipitation from acetone (PVDF, PNpSS, and PNpSS-b-PVDF-b-PNpSS) or direct dissolution in water (PNaSS-b-PVDF-b-PNaSS).
Remarkably, while the macroinitiator and the protected polymers were not stable in PBS solutions, all PNaSS-b-PVDF-b-PNaSS triblock copolymers provided indefinitely stable, water soluble nanoparticles even under the high ionic strength of PBS, modelling cell-isotonic conditions with pH = 7.4. These trends were found consistent with the dependence of Rh, conductivity, and zeta potential on composition where Rh increases upon deprotection from about 35–25 nm to about 168–136 nm in water, and decreases to 133–86 nm in PBS solutions, ζ decreases from ∼−40 mV to ∼−70 mV in water and increases to ∼−18 mV in PBS, while the conductivity is initially negligible, then increases linearly to ∼0.2 mS cm−1 for PNaSS-b-PVDF-b-PNaSS in water to reach ∼17 mS cm−1 in PBS.
While such blocks could find applications in fuel cells or non-fouling coatings, since both PNaSS and PVDF are already approved by FDA (U.S. Food and Drug Administration), the stability of their blocks under biologically relevant conditions can open up novel possibilities for their in vivo use, including drug carriers or contrast agents for imaging techniques.
Experimental section
Chemicals
Neopentyl p-styrenesulfonate (NpSS) was synthesized as described in the literature.22 Manganese(0) carbonyl (Mn2(CO)10), vinylidene fluoride (VDF), dimethyl carbonate (DMC), N,N-dimethylacetamide (DMAC), phosphate buffered saline in tablets (PBS; giving 0.01 M phosphate buffer of 0.0027 M potassium chloride and 0.137 M sodium chloride with pH 7.4 at 25 °C), nonafluoro-1-iodobutane (PFBI) and sodium azide (NaN3) were supplied by Sigma-Aldrich. DMC and DMAC were kept over molecular sieve (3 Å). 1,6-Diiodododecafluorohexane (DIPFH) was obtained from Matrix Scientific. Acetone, tetrahydrofuran (THF), diethyl ether, methanol, hydrochloric acid (HCl) and dimethyl sulfoxide (DMSO) were obtained from Lachner, Czech Republic. The water used at preparation of nanoparticles was pretreated with the Milli-Q® Plus System (Millipore Corporation). The dialysis membranes were obtained from Spectra/Por, USA.
Instrumental methods
1H NMR spectra (300 MHz) were obtained on a Bruker Advance DPX 300 NMR spectrometer with DMSO-d6 or acetone-d6 respectively as the solvents at 25 °C. The chemical shifts are relative to TMS using hexamethyldisiloxane (HMDSO, 0.05 from TMS in 1H NMR spectra) as internal standard. 19F NMR (400 MHz) spectra were recorded on a Bruker DRX-400 at 25 °C in acetone-d6.
Gel permeation chromatography (GPC) was performed on a calc 100 (Labio, Czech Republic) chromatograph equipped with a differential refractometer and a PLgel mixed-C column (Polymer Laboratories, UK) using DMAC as the eluent at 30 °C and at the flow rate of 0.70 mL min−1.
Water solutions were investigated by dynamic light scattering (DLS) using an ALV-6010 correlator (ALV GmbH, Germany) equipped with an ALV/CGS-8F goniometer, a 22 mW He–Ne laser (wavelength λ = 632.8 nm) and pair of avalanche photodiodes operated in a pseudo-cross-correlation mode. All measurements were made at a 90° angle. The measured intensity correlation function g2(t) was analyzed using the algorithm REPES67 performing the inverse Laplace transformation according to
|
 | (1) |
(where
t is the delay time of the correlation function and
β an instrumental parameter) and yielding distribution
A(
τ) of relaxation times
τ. The relaxation time
τ is related to the diffusion coefficient
D and relaxation (decay) rate
Γ by the relation
where
q is the scattering vector defined as
q = (4π
n/
λ)sin(
θ/2) where
n is the refractive index of the solvent and
θ is the scattering angle.
The particle apparent hydrodynamic radius Rh was calculated from the diffusion coefficient using the Stokes–Einstein equation:
where
T is the absolute temperature,
η the viscosity of the solvent and
kB the Boltzmann constant.
Electrophoretic (ELS) light scattering and conductivity measurements were performed using a Zetasizer NanoZS apparatus (Malvern Instruments, UK). The nanoparticle average zeta potential (ζ) was determined by measuring their electrophoretic mobility (UE) followed by conversion to ζ-potential (mV) through the Henry's equation:
where
ε is the dielectric constant of the medium. The parameter
f(
ka) is the Henry's function which has been calculated using the Smoluchowski approximation of
f(
ka) = 1.5.
Polymer synthesis
Synthesis and deprotection of poly(neopentyl styrenesulfonate) (PNpSS). A Schlenk tube containing a magnetic stirrer, NpSS (300 mg, 1.18 mmol, 20 eq.), Mn2(CO)10 (11 mg, 0.029 mmol, 0.5 eq.), 20.4 mg (0.059 mmol, 1 eq.) of PFBI and DMAC (1 mL) was degassed with Ar and heated at 90 °C for 19 h under visible light irradiation.22 The mixture was then precipitated into diethyl ether, centrifuged, dissolved in THF and reprecipitated. The product was thoroughly washed with diethyl ether and dried in vacuo to afford PNpSS, 225 mg, 75% yield. GPC: Mw = 8400 g mol−1, Mn = 7800 g mol−1, PDI = 1.19. Subsequently, PNpSS was deprotected as described before, using NaN3.22
Synthesis of the I–PVDF–I difunctional macroinitiator. The polymerization of the VDF was carried out in DMC, as we have previously described,22 using DIPFH as an initiator and [VDF]/[DIPFH]/[Mn2(CO)10] = 100/1/0.1, 40 °C, 21 h. The resulting iodine-terminated PVDF (I–PVDF60–I, 49.3% yield, Mw = 8.900 g mol−1, Mn = 6.200 g mol−1, PDI = 1.43. Mn (1H NMR) = 4400 g mol−1) was used as a bifunctional macroinitiator for the subsequent copolymerization. 1H and 19F-NMR analysis of the iodine chain ends indicates a total chain end functionality of at least 90%.
Synthesis of PNpSS-b-PVDF-b-PNpSS. A series of PNpSS-b-PVDF-b-PNpSS triblocks were prepared by variation of NpSS monomer content in the reaction mixture. These triblocks are denoted as T1, T2 and T3 in Tables 1 and 2 In a typical procedure, a Schlenk tube containing a magnetic stirrer and a mixture of NpSS (353 mg, 1.40 mmol, 155 eq.), I–PVDF60–I (50 mg, 0.009 mmol, 1 eq.), Mn2(CO)10 (7 mg, 0.018 mmol, 2 eq.) and 4 mL of DMAC was degassed with argon and placed into an oil bath preheated to 65 °C and irradiated with visible light as previously described22 for 48 hours. The mixture was then precipitated into methanol/water (2/1), centrifuged, dissolved in acetone and reprecipitated in methanol/water acidified with 2 M HCl. The product was thoroughly washed with methanol and dried in vacuo to afford PNpSS-b-PVDF-b-PNpSS, 226 mg, 56% yield. GPC: Mw = 25
600 g mol−1, Mn = 13
800 g mol−1, PDI = 1.86. 1H NMR composition: PNpSS34-b-PVDF60-b-PNpSS34, Mn (1H NMR) = 21
700 g mol−1.
Synthesis of PNaSS-b-PVDF-b-PNaSS. In a typical procedure,22 40 mg of the polymer, 10-fold excess of NaN3 (vs. NpSS) and 2 mL of DMSO were placed into a vial with a magnetic stirrer which was placed in an oil bath preheated to 120 °C and stirred for 10 hours. The reaction mixture was then diluted with 5 mL of distilled water, transferred into a dialysis bag (MWCO 2000 Da), dialyzed against deionized water for 5 days, and the product was isolated by lyophilisation. These triblocks are denoted as T1D, T2D and T3D in Table 2.
Preparation and characterization of nanoparticles
In the case of NpSS based triblock copolymers, PNpSS and I–PVDF–I, the nanoparticles (NP) were prepared by the nanoprecipitation method62 using acetone stock solution of the studied material with the concentration 2 mg mL−1. Typically, 2 mL of the stock solution was poured into 5 mL of Milli-Q® water using motor driven syringe with rate of 2 mL min−1, while stirring the system at 350 rpm. The organic phase was removed by evaporation at ambient temperature (rotary evaporator, 10 minutes), and the volume of the final solution was adjusted with water to 5 mL and a final polymer concentration of 0.4 mg mL−1.
Preliminary 19F MRI results were obtained with cooperation of Institute for Clinical and Experimental Medicine, Prague, Czech Republic using a preclinical MRI system.
Acknowledgements
Financial support provided by the Czech Ministry of Education, Youth and Sports (Grant # MSMT KONTAKT II LH14038) and the National Science Foundation grant CHEM-1309769 is gratefully acknowledged. We also gratefully acknowledge Daniel Jirak, Department of Diagnostic and Interventional Radiology, Institute for Clinical and Experimental Medicine, Prague, Czech Republic, for providing testing MRI phantom measurement (funds for development of research organization 00023001, IKEM, Prague, Czech Republic).
References
- Global Fluoropolymer Market Report 2013–2018: PTFE, PVDF, FEP, Fluoroelastomers in Fluoropolymer Market by Types, by Applications-Global Trends and Forecasts to 2019, Dublin, Ireland, 2015 Search PubMed
. - Chemical Economics Handbook Fluoropolymers, IHS Inc, Colorado, Englewood, Colorado, 2012 Search PubMed
. - B. Ameduri, Macromolecules, 2010, 43, 10163–10184 CrossRef CAS
. - B. Ameduri, Chem. Rev., 2009, 109, 6632–6686 CrossRef CAS PubMed
. - A. Alaaeddine, J. Vergnaud, J. Rolland, A. Vlad, J.-F. Gohy and B. Ameduri, Polym. Chem., 2015, 6, 6021–6028 RSC
. - F.-C. Sun, A. M. Dongare, A. D. Asandei, S. P. Alpay and S. Nakhmanson, J. Mater. Chem. C, 2015, 3, 8389–8396 RSC
. - B. Ameduri and B. Boutevin, in Well-Architectured Fluoropolymers: Synthesis, Properties and Applications, Elsevier, 2004, pp. 1–99 Search PubMed
. - L. Zhu, J. Phys. Chem. Lett., 2014, 5, 3677–3687 CrossRef CAS PubMed
. - J. E. Dohany, Fluorine-Containing Polymers, Poly(Vinylidene Fluoride), John Wiley & Sons, Inc, Hoboken, NJ, USA, 2000 Search PubMed
. - A. D. Asandei, Chem. Rev., 2016, 116, 2244–2274 CrossRef CAS PubMed
. - N. M. L. Hansen, K. Jankova and S. Hvilsted, Eur. Polym. J., 2007, 43, 255–293 CrossRef CAS
. - K. Matyjaszewski, Macromolecules, 2012, 45, 4015–4039 CrossRef CAS
. - B. Ameduri, J. Taiwan Inst. Chem. Eng., 2014, 45, 3124–3133 CrossRef CAS
. - G. David, C. Boyer, J. Tonnar, B. Ameduri, P. Lacroix-Desmazes and B. Boutevin, Chem. Rev., 2006, 106, 3936–3962 CrossRef CAS PubMed
. - P. Lacroix-Desmazes and J. Tonnar, in Polymer Science: A Comprehensive Reference, ed. K. Matyjaszewski and M. Möller, Elsevier, 2012, vol. 3, pp. 159–180 Search PubMed
. - M. Oka and M. Tatemoto, in Contemporary Topics in Polymer Science, ed. W. J. Bailey and T. Tsuruta, Springer, New York, Boston, MA, 1984, vol. 4, pp. 763–777 Search PubMed
. - G. Laruelle, E. Nicol, B. Ameduri, J.-F. Tassin and N. Ajellal, J. Polym. Sci., Part A: Polym. Chem., 2011, 49, 3960–3969 CrossRef CAS
. - A. D. Asandei, C. P. Simpson, O. I. Adebolu and Y. Chen, in Advances in Fluorine-Containing Polymers, ed. D. W. Smith, S. T. Iacono, D. J. Boday and S. C. Kettwich, American Chemical Society, 2012, vol. 1106, pp. 47–63 Search PubMed
. - A. D. Asandei, O. I. Adebolu and C. P. Simpson, J. Am. Chem. Soc., 2012, 134, 6080–6083 CrossRef CAS PubMed
. - A. D. Asandei, O. I. Adebolu and C. P. Simpson, in Handbook of Fluoropolymer Science and Technology, ed. D. W. Smith, S. T. Iacono and S. S. Iyer, John Wiley & Sons, Inc, Hoboken, NJ, USA, 2014, pp. 21–42 Search PubMed
. - A. D. Asandei, O. I. Adebolu, C. P. Simpson and J.-S. Kim, Angew. Chem., Int. Ed. Engl., 2013, 52, 10027–10030 CrossRef CAS PubMed
. - P. Černoch, S. Petrova, Z. Černochová, J.-S. Kim, C. P. Simpson and A. D. Asandei, Eur. Polym. J., 2015, 68, 460–470 CrossRef
. - C. P. Simpson, O. I. Adebolu, J.-S. Kim, V. Vasu and A. D. Asandei, Macromolecules, 2015, 48, 6404–6420 CrossRef CAS
. - C. P. Simpson, O. I. Adebolu, J.-S. Kim, V. Vasu and A. D. Asandei, in Controlled Radical Polymerization: Mechanisms, ed. K. Matyjaszewski, B. S. Sumerlin, N. V. Tsarevsky and J. Chiefari, American Chemical Society, 2015, vol. 1187, pp. 183–209 Search PubMed
. - A. D. Asandei, Patent US9193810 B2, 2015
. - A. D. Asandei, C. P. Simpson, O. I. Adebolu and Y. Chen, Polym. Prepr., 2011, 52, 728–729 CAS
. - F. di Lena and K. Matyjaszewski, Prog. Polym. Sci., 2010, 35, 959–1021 CrossRef CAS
. -
(a) A. D. Asandei and Y. Chen, Polym. Mater. Sci. Eng., 2007, 97, 450 CAS
;
(b) A. D. Asandei and G. Saha, Polym. Prepr., 2007, 48, 272 CAS
;
(c) A. D. Asandei and Y. Chen, Macromolecules, 2006, 39, 7549 CrossRef CAS
;
(d) A. D. Asandei, et al., J. Organomet. Chem., 2007, 692, 3174 CrossRef CAS
;
(e) A. D. Asandei, et al., Tetrahedron, 2008, 64, 11831 CrossRef CAS
;
(f) A. D. Asandei and I. W. Moran, J. Polym. Sci., Part A: Polym. Chem., 2006, 44, 1060 CrossRef CAS
;
(g) A. D. Asandei, et al., J. Polym. Sci., Part A: Polym. Chem., 2006, 44, 2156 CrossRef CAS
;
(h) A. D. Asandei, et al., J. Polym. Sci., Part A: Polym. Chem., 2006, 44, 2015 CrossRef CAS
;
(i) A. D. Asandei and G. Saha, J. Polym. Sci., Part A: Polym. Chem., 2006, 44, 1106 CrossRef CAS
;
(j) A. D. Asandei and I. W. Moran, J. Am. Chem. Soc., 2004, 126, 15932 CrossRef CAS PubMed
;
(k) A. D. Asandei and I. W. Moran, J. Polym. Sci., Part A: Polym. Chem., 2005, 43, 6028 CrossRef CAS
;
(l) A. D. Asandei and I. W. Moran, J. Polym. Sci., Part A: Polym. Chem., 2005, 43, 6039 CrossRef CAS
. - A. D. Asandei, I. W. Moran, G. Saha and Y. Chen, in Controlled/Living Radical Polymerization, ed. K. Matyjaszewski, American Chemical Society, 2006, vol. 944, pp. 125–139 Search PubMed
. -
(a) A. D. Asandei and G. Saha, Polym. Prepr., 2005, 46, 474 CAS
;
(b) A. D. Asandei and C. P. Simpson, Polym. Prepr., 2008, 49, 75 CAS
. - A. D. Asandei, C. P. Simpson, S. Y. Hyun, O. I. Adebolu, G. Saha and Y. Chen, in Controlled/Living Radical Polymerization: Progress in RAFT, DT, NMP & OMRP, ed. K. Matyjaszewski, American Chemical Society, Washington, DC, 2009, vol. 1024, pp. 149–163 Search PubMed
. - A. D. Asandei, Y. Chen, O. I. Adebolu and C. P. Simpson, J. Polym. Sci., Part A: Polym. Chem., 2008, 46, 2869–2877 CrossRef CAS
. - A. D. Asandei and G. Saha, Macromol. Rapid Commun., 2005, 26, 626–631 CrossRef CAS
. - A. D. Asandei, G. Saha and Y. Chen, Polym. Prepr., 2005, 46, 848–849 CAS
. -
(a) A. D. Asandei and G. Saha, Macromolecules, 2006, 39, 8999 CrossRef CAS
;
(b) A. D. Asandei and G. Saha, Polym. Prepr., 2007, 48, 308 CAS
;
(c) A. D. Asandei and G. Saha, Polym. Prepr., 2007, 48, 381 CAS
;
(d) A. D. Asandei and G. Saha, Polym. Mater. Sci. Eng., 2007, 96, 435 CAS
;
(e) A. D. Asandei and G. Saha, Polym. Mater. Sci. Eng., 2005, 93, 663 Search PubMed
;
(f) A. D. Asandei, et al., Mater. Res. Soc. Symp. Proc., 2005, 856, BB11 Search PubMed
;
(g) A. D. Asandei, et al., Polym. Mater. Sci. Eng., 2004, 91, 786 CAS
;
(h) A. D. Asandei, et al., Polym. Mater. Sci. Eng., 2006, 95, 569 CAS
;
(i) A. D. Asandei and G. Saha, Polym. Mater. Sci. Eng., 2006, 95, 573 CAS
;
(j) A. D. Asandei and G. Saha, Polym. Prepr., 2006, 47, 831 CAS
;
(k) A. D. Asandei, G. Saha and A. Ranade, Polym. Prepr., 2006, 47, 501 CAS
;
(l) A. D. Asandei and G. Saha, Polym. Prepr., 2004, 45, 768 CAS
;
(m) A. D. Asandei and G. Saha, Polym. Prepr., 2005, 46, 674–675 CAS
. - A. D. Asandei and G. Saha, Polym. Prepr., 2007, 48, 274–275 CAS
. - E. T. Ahrens and J. Zhong, NMR Biomed., 2013, 26, 860–871 CrossRef CAS PubMed
. - K. H. Chalmers, E. De Luca, N. H. M. Hogg, A. M. Kenwright, I. Kuprov, D. Parker, M. Botta, J. I. Wilson and A. M. Blamire, Chem.–Eur. J., 2010, 16, 134–148 CrossRef CAS PubMed
. - I. Tirotta, A. Mastropietro, C. Cordiglieri, L. Gazzera, F. Baggi, G. Baselli, M. G. Bruzzone, I. Zucca, G. Cavallo, G. Terraneo, F. Baldelli Bombelli, P. Metrangolo and G. Resnati, J. Am. Chem. Soc., 2014, 136, 8524–8527 CrossRef CAS PubMed
. - K. Wang, H. Peng, K. J. Thurecht, S. Puttick and A. K. Whittaker, Polym. Chem., 2016, 7, 1059–1069 RSC
. - W. Du, A. M. Nyström, L. Zhang, K. T. Powell, Y. Li, C. Cheng, S. A. Wickline and K. L. Wooley, Biomacromolecules, 2008, 9, 2826–2833 CrossRef CAS PubMed
. - I. Tirotta, V. Dichiarante, C. Pigliacelli, G. Cavallo, G. Terraneo, F. B. Bombelli, P. Metrangolo and G. Resnati, Chem. Rev., 2015, 115, 1106–1129 CrossRef CAS PubMed
. - C. Santaella, F. Frézard, P. Vierling and J. G. Riess, FEBS Lett., 1993, 336, 481–484 CrossRef CAS PubMed
. - R. Dembinski, R. Bensberg, G. Marx, R. Rossaint, M. Quintel and R. Kuhlen, Exp. Lung Res., 2010, 36, 499–507 CrossRef CAS PubMed
. - P. Vierling, C. Santaella and J. Greiner, J. Fluorine Chem., 2001, 107, 337–354 CrossRef CAS
. - J. G. Riess, Tetrahedron, 2002, 58, 4113–4131 CrossRef CAS
. - C. M. Friesen and B. Ameduri, Macromolecules, 2015, 48, 7060–7070 CrossRef CAS
. - M. N. Wadekar, Y. R. Patil and B. Ameduri, Macromolecules, 2014, 47, 13–25 CrossRef CAS
. - J. Walkowiak-Kulikowska, A. Szwajca, F. Boschet, V. Gouverneur and B. Ameduri, Macromolecules, 2014, 47, 8634–8644 CrossRef CAS
. - K. Matsumoto, H. Mazaki and H. Matsuoka, Macromolecules, 2004, 37, 2256–2267 CrossRef CAS
. - G. Tae, J. a. Kornfield, J. a. Hubbell, D. Johannsmann and T. E. Hogen-Esch, Macromolecules, 2001, 34, 6409–6419 CrossRef CAS
. - R. Kaplánek, O. Paleta, I. Ferjentsiková and M. Kodíček, J. Fluorine Chem., 2009, 130, 308–316 CrossRef
. - J. N. Slaughter, K. M. Schmidt, J. L. Byram and S. Mecozzi, Tetrahedron Lett., 2007, 48, 3879–3882 CrossRef CAS
. - L. Le Gourriérec, C. Di Giorgio, J. Greiner and P. Vierling, New J. Chem., 2008, 32, 2027–2042 RSC
. - S. Xu and W. Liu, J. Polym. Sci., Part B: Polym. Phys., 2008, 46, 1000–1006 CrossRef CAS
. - R. Labruère and E. Turos, Bioorg. Med. Chem., 2012, 20, 5042–5045 CrossRef PubMed
. - Q. Peng, S. Lu, D. Chen, X. Wu, P. Fan, R. Zhong and Y. Xu, Macromol. Biosci., 2007, 7, 1149–1159 CrossRef CAS PubMed
. - L. Ying, W. H. Yu, E. T. Kang and K. G. Neoh, Langmuir, 2004, 20, 6032–6040 CrossRef CAS PubMed
. - Y. Chen, L. Ying, W. Yu, E. T. Kang and K. G. Neoh, Macromolecules, 2003, 36, 9451–9457 CrossRef CAS
. - Q. Berrod, S. Lyonnard, A. Guillermo, J. Ollivier, B. Frick, A. Manseri, B. Améduri and G. Gébel, Macromolecules, 2015, 48, 6166–6176 CrossRef CAS
. - F. S. Bates and G. H. Fredrickson, Phys. Today, 1999, 52, 32 CrossRef CAS
. - A. M. de Oliveira, E. Jäger, A. Jäger, P. Stepánek and F. C. Giacomelli, Colloids Surf., A, 2013, 436, 1092–1102 CrossRef CAS
. - M. A. Villetti, R. Borsali, J. S. Crespo, V. Soldi and K. Fukada, Macromol. Chem. Phys., 2004, 205, 907–917 CrossRef CAS
. - M. Karayianni, G. Mountrichas and S. Pispas, J. Phys. Chem. B, 2010, 114, 10748–10755 CrossRef CAS PubMed
. - J.-M. Y. Carrillo and A. V Dobrynin, Macromolecules, 2011, 44, 5798–5816 CrossRef CAS
. - A. Dobrynin and M. Rubinstein, Prog. Polym. Sci., 2005, 30, 1049–1118 CrossRef CAS
. - J. Jakeš, Collect. Czech. Chem. Commun., 1995, 60, 1781–1797 CrossRef
.
|
This journal is © The Royal Society of Chemistry 2016 |